Chapter 18 Geological Resources
Adapted from Physical Geology, First University of Saskatchewan Edition and Physical Geology (Steven Earle)
Learning Objectives
After reading this chapter, completing the exercises within it, and answering the questions at the end, you should be able to:
- Describe the importance of geological resources to our way of life
- Summarize the types of materials mined in Canada and explain some of the processes involved in the formation of metal deposits
- Explain how a metal deposit is developed into a mine
- Define acid rock drainage (ARD) and discuss why some mines can lead to ARD and contamination of the environment by metals
- Summarize some of the important industrial materials extracted in Canada and describe what they are used for
- Describe the processes that lead to the formation of coal deposits
- Explain the processes that lead to the formation of oil and gas, the distinction between source rocks and reservoir rocks, and the importance of traps
- Describe the origins and recovery of some of the unconventional fossil fuels
- Describe the origins, discovery, and extraction of diamonds in Canada
18.1 If You Can’t Grow It, You Have to Mine It
It has been said that “if you can’t grow it, you have to mine it,” meaning that anything we can’t grow we have to extract from Earth in one way or another. This includes water, of course, our most important resource, but it also includes all the other materials that we need to construct things like roads, dams, and bridges, or manufacture things like plates, toasters, and telephones. Even most of our energy resources come from Earth, including uranium and fossil fuels, and much of the infrastructure of this electrical age depends on copper (Figure 18.1).
![The open pit (background) and waste-rock piles (middle) of the Highland Valley Copper Mine at Logan Lake, British Columbia [Photo by Russell Hartlaub, used with permission]](figures/18-geological-resources/figure-18-1.jpg)
Figure 18.1: The open pit (background) and waste-rock piles (middle) of the Highland Valley Copper Mine at Logan Lake, British Columbia [Photo by Russell Hartlaub, used with permission]
Virtually everything we use every day is made from resources from Earth. For example, let’s look at a tablet computer (Figure 18.2). Most of the case is made of a plastic known as ABS, which is made from either gas or petroleum. Some tablets have a case made from aluminum. The glass of a touch screen is made mostly from quartz combined with smaller amounts of sodium oxide (Na2O), sodium carbonate (Na2CO3), and calcium oxide (CaO). To make it work as a touch screen, the upper surface is coated with indium tin oxide. When you touch the screen you’re actually pushing a thin layer of polycarbonate plastic (made from petroleum) against the coated glass — completing an electrical circuit. The computer is then able to figure out exactly where you touched the screen. Computer processors are made from silica wafers (more quartz) and also include a significant amount of copper and gold. Gold is used because it is a better conductor than copper and doesn’t tarnish the way silver or copper does. Most computers have nickel-metal-hydride (NiMH) batteries, which contain nickel, of course, along with cadmium, cobalt, manganese, aluminum, and the rare-earth elements lanthanum, cerium, neodymium, and praseodymium. The processor and other electronic components are secured to a circuit board, which is a thin layer of fibreglass sandwiched between copper sheets coated with small amounts of tin and lead. Various parts are put together with steel screws that are made of iron and molybdenum.
![The main components of a tablet computer [SE, base photograph from https://upload.wikimedia.org/wikipedia/commons/8/8d/IPad_Air.png]](figures/18-geological-resources/figure-18-2.png)
Figure 18.2: The main components of a tablet computer [SE, base photograph from https://upload.wikimedia.org/wikipedia/commons/8/8d/IPad_Air.png]
That’s not everything that goes into a tablet computer, but to make just those components we need a pure-silica sand deposit, a salt mine for sodium, a rock quarry for calcium, an oil well, a gas well, an aluminum mine, an iron mine, a manganese mine, a copper-molybdenum-gold mine, a cobalt-nickel mine, a rare-earth element and indium mine, and a source of energy to transport all of the materials, process them, put them together, and finally transport the computer to your house or the store where you bought it.
Exercise: Where Does It Come From?
Look around you and find at least five objects (other than a computer or a phone) that have been made from materials that had to be mined, quarried, or extracted from an oil or gas well. Try to identify the materials involved, and think about where they might have come from. This pen is just an example.
18.2 Metal Deposits
Mining has always been a major part of Canada’s economy. Canada has some of the largest mining districts and deposits in the world, and for the past 150 years, we have been one of the most important suppliers of metals. Extraction of Earth’s resources goes back a long way in Canada. For example, the First Nations of British Columbia extracted obsidian from volcanic regions for tools and traded it up and down the coast. In the 1850s, gold was discovered in central British Columbia, and in the 1890s, even more gold was discovered in the Klondike area of Yukon. These two events were critical to the early development of British Columbia, Yukon, and Alaska.
Canada’s mining sector had revenues in the order of $37 billion in 2013. The majority of that was split roughly equally among gold, iron, copper, and potash, with important but lesser amounts from nickel and diamonds (Figure 18.4). Revenues from the petroleum sector are significantly higher, at over $100 billion per year.
![The value of various Canadian mining sectors in 2013 [SE from data at http://www.nrcan.gc.ca/mining-materials/publications/8772]](figures/18-geological-resources/figure-18-3.png)
Figure 18.4: The value of various Canadian mining sectors in 2013 [SE from data at http://www.nrcan.gc.ca/mining-materials/publications/8772]
A metal deposit is a body of rock in which one or more metals have been concentrated to the point of being economically viable for recovery. Some background levels of important metals in average rocks are shown on Table 18.1, along with the typical grades necessary to make a viable deposit, and the corresponding concentration factors. Looking at copper, for example, we can see that while average rock has around 40 ppm (parts per million) of copper, a grade of around 10,000 ppm or 1% is necessary to make a viable copper deposit. In other words, copper ore has about 250 times as much copper as typical rock. For the other elements in the list, the concentration factors are much higher. For gold, it’s 2,000 times and for silver it’s around 10,000 times.
Metal | Typical Background Level | Typical Economic Grade | Concentration Factor |
---|---|---|---|
Copper | 40 ppm | 10,000 ppm (1%) | 250 times |
Gold | 0.003 ppm | 6 ppm (0.006%) | 2,000 times |
Lead | 10 ppm | 50,000 ppm (5% | 5,000 times |
Molybdenum | 1 ppm | 1,000 ppm (0.1%) | 1,000 times |
Nickel | 25 ppm | 20,000 ppm (2%) | 800 times |
Silver | 0.1 ppm | 1,000 ppm (0.1%) | 10,000 times |
Uranium | 2 ppm | 10,000 ppm (1%) | 5,000 times |
Zinc | 50 ppm | 50,000 ppm (5%) | 1,000 times |
It is clear that some very significant concentration must take place to form a mineable deposit. This concentration may occur during the formation of the host rock, or after the rock forms, through a number of different types of processes. There is a very wide variety of ore-forming processes, and there are hundreds of types of mineral deposits. The origins of a few of them are described below.
18.2.1 Types of Metal Deposits
18.2.1.1 Magmatic Nickel Deposits
A magmatic deposit is one in which the metal concentration takes place primarily at the same time as the formation and emplacement of the magma. Most of the nickel mined in Canada comes from magmatic deposits such as those in Sudbury (Ontario), Thompson (Manitoba) (Figure 18.5), and Voisey’s Bay (Labrador). The magmas from which these deposits form are of mafic or ultramafic composition (derived from the mantle), and therefore they have relatively high nickel and copper contents to begin with (as much as 100 times more than normal rocks in the case of nickel). These elements may be further concentrated within the magma as a result of the addition of sulphur from partial melting of the surrounding rocks. The heavy nickel and copper sulphide minerals are then concentrated further still by gravity segregation (i.e., crystals settling toward the bottom of the magma chamber). In some cases, there are significant concentrations of platinum-bearing minerals.
Most of these types of deposits around the world are Precambrian in age — probably because the mantle was significantly hotter at that time, and the necessary mafic and ultramafic magmas were more likely to be emplaced in the continental crust.
![The nickel smelter at Thompson, Manitoba [https://en.wikipedia.org/wiki/Thompson,_Manitoba#/media/File:Vale_Nickel_Mine.JPG]](figures/18-geological-resources/figure-18-4.jpg)
Figure 18.5: The nickel smelter at Thompson, Manitoba [https://en.wikipedia.org/wiki/Thompson,_Manitoba#/media/File:Vale_Nickel_Mine.JPG]
18.2.1.2 Volcanogenic Massive Sulphide Deposits
Much of the copper, zinc, lead, silver, and gold mined in Canada is mined from volcanic-hosted massive sulphide (VHMS) deposits associated with submarine volcanism (VMS deposits). Examples are the deposits at Kidd Creek, Ontario, Flin Flon on the Manitoba-Saskatchewan border, Britannia on Howe Sound, and Myra Falls (within Strathcona Park) on Vancouver Island.
VMS deposits are formed from the water discharged at high temperature (250° to 300°C) at ocean-floor hydrothermal vents, primarily in areas of subduction-zone volcanism. The environment is comparable to that of modern-day black smokers (Figure 18.6), which form where hot metal- and sulphide-rich water issues from the sea floor. They are called massive sulphide deposits because the sulphide minerals (including pyrite (FeS2) , sphalerite (ZnS), chalcopyrite (CuFeS2), and galena (PbS)) are generally present in very high concentrations (making up the majority of the rock in some cases). The metals and the sulphur are leached out of the sea-floor rocks by convecting groundwater driven by the volcanic heat, and then quickly precipitated where that hot water enters the cold seawater, causing it to cool suddenly and change chemically. The volcanic rock that hosts the deposits is formed in the same area and at the same general time as the accumulation of the ore minerals.
![Left: A black smoker on the Juan de Fuca Ridge off the west coast of Vancouver Island. Right: A model of the formation of a volcanogenic massive sulphide deposit on the sea floor. [left: NOAA at: http://oceanexplorer.noaa.gov/okeanos/explorations/10index/background/plumes/media/black_smoker.html, right: SE]](figures/18-geological-resources/figure-18-5.png)
Figure 18.6: Left: A black smoker on the Juan de Fuca Ridge off the west coast of Vancouver Island. Right: A model of the formation of a volcanogenic massive sulphide deposit on the sea floor. [left: NOAA at: http://oceanexplorer.noaa.gov/okeanos/explorations/10index/background/plumes/media/black_smoker.html, right: SE]
18.2.2 Porphyry Deposits
Porphyry deposits are the most important source of copper and molybdenum in British Columbia, the western United States, and Central and South America. Most porphyry deposits also host some gold, which may be, in rare cases, the primary commodity. B.C. examples include several large deposits within the Highland Valley mine (Figure 18.1) and numerous other deposits scattered around the central part of the province.
A porphyry deposit forms around a cooling felsicstock in the upper part of the crust. They are called “porphyry” because upper crustal stocks are typically porphyritic in texture, the result of a two-stage cooling process. Metal enrichment results in part from convection of groundwater related to the heat of the stock, and also from metal-rich hot water expelled by the cooling magma (Figure 18.7). The host rocks, which commonly include the stock itself and the surrounding country rocks, are normally highly fractured and brecciated. During the ore-forming process, some of the original minerals in these rocks are altered to potassium feldspar, biotite, epidote, and various clay minerals. The important ore minerals include chalcopyrite (CuFeS2), bornite (Cu5FeS4), and pyrite in copper porphyry deposits, or molybdenite (MoS2) and pyrite in molybdenum porphyry deposits. Gold is present as minute flakes of native gold.
This type of environment (i.e., around and above an intrusive body) is also favourable for the formation of other types of deposits — particularly vein-type gold deposits (a.k.a. epithermal deposits). Some of the gold deposits of British Columbia (such as in the Eskay Creek area adjacent to the Alaska panhandle), and many of the other gold deposits situated along the western edge of both South and North America are of the vein type shown in Figure 18.7, and are related to nearby magma bodies.
![A model for the formation of a porphyry deposit around an upper-crustal porphyritic stock and associated vein deposits. [SE]](figures/18-geological-resources/figure-18-6.png)
Figure 18.7: A model for the formation of a porphyry deposit around an upper-crustal porphyritic stock and associated vein deposits. [SE]
18.2.2.1 Banded Iron Formation
Most of the world’s major iron deposits are of the banded iron formation type (classified as a type of chemical sedimentary rock), and most of these formed during the initial oxygenation of Earth’s atmosphere between 2,400 and 1,800 Ma. At that time, iron that was present in dissolved form in the ocean (as Fe2+) became oxidized to its insoluble form (Fe3+) and accumulated on the sea floor, mostly as hematite interbedded with chert (Figure 18.8). Unlike many other metals, which are economically viable at grades of around 1% or even much less, iron deposits are only viable if the grades are in the order of 50% iron and if they are very large.
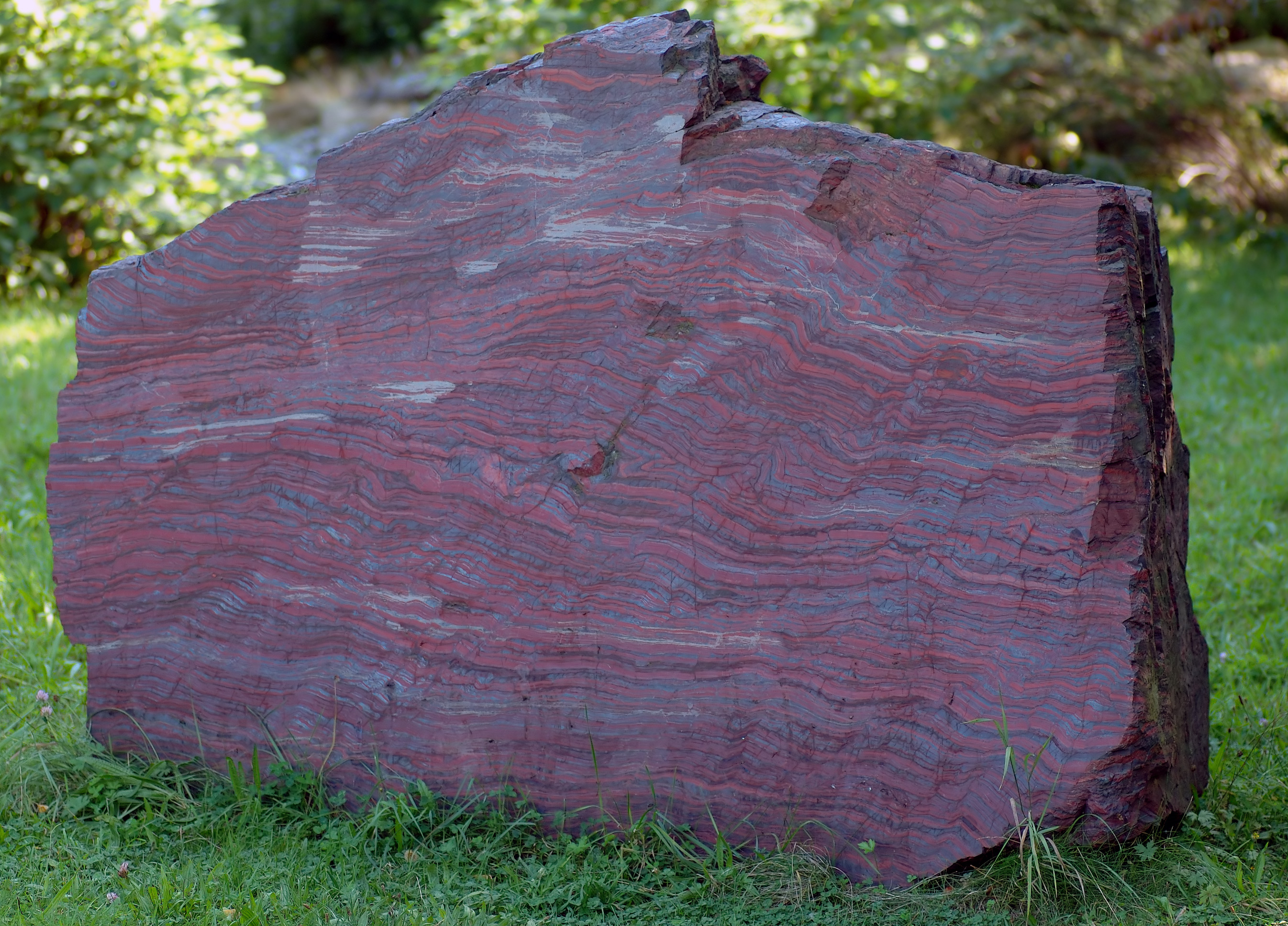
Figure 18.8: Banded iron formation from an unknown location in North America on display at a museum in Germany. The rock is about 2 m across. The dark grey layers are magnetite and the red layers are hematite. Chert is also present. Source: https://upload.wikimedia.org/wikipedia/commons/5/5f/Black-band_ironstone_%28aka%29.jpg
18.2.2.2 Unconformity-Type Uranium Deposits
![Model of the formation of unconformity-type uranium deposits of the Athabasca Basin, Saskatchewan [SE]](figures/18-geological-resources/figure-18-8.png)
Figure 18.9: Model of the formation of unconformity-type uranium deposits of the Athabasca Basin, Saskatchewan [SE]
There are several different types of uranium deposits, but some of the largest and richest are those within the Athabasca Basin of northern Saskatchewan. These are called unconformity-type uranium deposits because they are all situated very close to the unconformity between the Proterozoic Athabasca Group sandstone and the much older Archean sedimentary, volcanic, and intrusive igneous rock (Figure 18.9). The origin of unconformity-type U deposits is not perfectly understood, but it is thought that two particular features are important: (1) the relative permeability of the Athabasca Group sandstone, and (2) the presence of graphitic schist within the underlying Archean rocks. The permeability of the sandstone allowed groundwater to flow through it and leach out small amounts of U, which stayed in solution in the oxidized form U6+. The graphite (C) created a reducing environment (non-oxidizing) that converted the U from U6+ to insoluble U4+, at which point it was precipitated as the mineral uraninite (UO2).
Exercise: The Importance of Heat and Heat Engines
For a variety of reasons, thermal energy (heat) from within Earth is critical in the formation of many types of ore deposits. Look back through the deposit-type descriptions above and complete the following table, describing (1) which of those deposit types depend on a source of heat for their formation, and (2) if so, why?
Magmatic
Volcanogenic massive sulphide
Porphyry
Banded iron formation
Unconformity-type uranium
18.3 Mining and Mineral Processing
Metal deposits are mined in a variety of different ways depending on their depth, shape, size, and grade. Relatively large deposits that are quite close to the surface and somewhat regular in shape are mined using open-pit mine methods (Figure 18.1). Creating a giant hole in the ground is generally cheaper than making an underground mine, but it is also less precise, so it is necessary to mine a lot of waste rock along with the ore. Relatively deep deposits or those with elongated or irregular shapes are typically mined from underground with deep vertical shafts, declines (sloped tunnels), and levels (horizontal tunnels) (Figures 18.10 and 18.11). In this way, it is possible to focus the mining on the ore body itself. However, with relatively large ore bodies, it may be necessary to leave some pillars to hold up the roof.
![Underground at the Myra Falls Mine, Vancouver Island. [SE]](figures/18-geological-resources/figure-18-9.jpg)
Figure 18.10: Underground at the Myra Falls Mine, Vancouver Island. [SE]
![Schematic cross-section of a typical underground mine. [SE]](figures/18-geological-resources/figure-18-10.png)
Figure 18.11: Schematic cross-section of a typical underground mine. [SE]
In many cases, the near-surface part of an ore body is mined with an open pit, while the deeper parts are mined underground (Figures 18.11 and 18.12).
![Entrance to an exploratory decline (white arrow) for the New Afton Mine situated in the side of the open pit of the old Afton Mine, near Kamloops, B.C. [SE]](figures/18-geological-resources/figure-18-11.png)
Figure 18.12: Entrance to an exploratory decline (white arrow) for the New Afton Mine situated in the side of the open pit of the old Afton Mine, near Kamloops, B.C. [SE]
A typical metal deposit might contain a few percent of ore minerals (e.g., chalcopyrite or sphalerite), mixed with the minerals of the original rock (e.g., quartz or feldspar). Other sulphide minerals are commonly present within the ore, especially pyrite.
When ore is processed (typically very close to the mine), it is ground to a fine powder and the ore minerals are physically separated from the rest of the rock to make a concentrate. At a molybdenum mine, for example, this concentrate may be almost pure molybdenite (MoS2). The rest of the rock is known as tailings. It comes out of the concentrator as a wet slurry and must be stored near the mine, in most cases, in a tailings pond.
The tailings pond at the Myra Falls Mine on Vancouver Island is shown in Figure 18.13, and the settling ponds for waste water from the concentrator are shown in Figure 18.14. The tailings are contained by an embankment. Also visible in the foreground of Figure 18.13 is a pile of waste rock, which is non-ore rock that was mined in order to access the ore. Although this waste rock contains little or no ore minerals, at many mines it contains up to a few percent pyrite. The tailings and the waste rock at most mines are an environmental liability because they contain pyrite plus small amounts of ore minerals. When pyrite is exposed to oxygen and water, it generates sulphuric acid — also known as acid rock drainage (ARD). Acidity itself is a problem to the environment, but because the ore elements, such as copper or lead, are more soluble in acidic water than neutral water, ARD is also typically quite rich in metals, many of which are toxic.
![The tailings pond at the Myra Falls Mine on Vancouver Island. The dry rock in the middle of the image is waste rock. The structure on the right is the headframe for the mine shaft. Myra Creek flows between the tailings pond and the headframe. [SE]](figures/18-geological-resources/figure-18-12.jpg)
Figure 18.13: The tailings pond at the Myra Falls Mine on Vancouver Island. The dry rock in the middle of the image is waste rock. The structure on the right is the headframe for the mine shaft. Myra Creek flows between the tailings pond and the headframe. [SE]
![The tailings pond (lower left) at Myra Falls Mine with settling ponds (right) for processing water from the concentrator. [SE]](figures/18-geological-resources/figure-18-13.jpg)
Figure 18.14: The tailings pond (lower left) at Myra Falls Mine with settling ponds (right) for processing water from the concentrator. [SE]
Tailings ponds and waste-rock storage piles must be carefully maintained to ensure their integrity and monitored to ensure that acidic and metal-rich water is not leaking out. In August 2014, the tailings pond at the Mt. Polley Mine in central B.C. failed and 10 million cubic metres of waste water along with 4.5 million cubic metres of tailings slurry was released into Polley Lake, Hazeltine Creek, and Quesnel Lake (Figure 18.15, 18.16). As of July 2015, the environmental implications of this event are still not fully understood.
![The Mt. Polley Mine area prior to the dam breach of August 2014. The tailings were stored in the area labelled "retention basin." [https://en.wikipedia.org/wiki/Mount_Polley_mine_disaster]](figures/18-geological-resources/figure-18-14a.jpg)
Figure 18.15: The Mt. Polley Mine area prior to the dam breach of August 2014. The tailings were stored in the area labelled “retention basin.” [https://en.wikipedia.org/wiki/Mount_Polley_mine_disaster]
![The Mt. Polley Mine area after the tailings dam breach of August 2014. The water and tailings released flowed into Hazeltine Creek, and Polley and Quesnel Lakes. [https://en.wikipedia.org/wiki/Mount_Polley_mine_disaster]](figures/18-geological-resources/figure-18-14b.jpg)
Figure 18.16: The Mt. Polley Mine area after the tailings dam breach of August 2014. The water and tailings released flowed into Hazeltine Creek, and Polley and Quesnel Lakes. [https://en.wikipedia.org/wiki/Mount_Polley_mine_disaster]
Most mines have concentrators on site because it is relatively simple to separate ore minerals from non-ore minerals and thus significantly reduce the costs and other implications of transportation. But separation of ore minerals is only the preliminary stage of metal refinement, for most metals the second stage involves separating the actual elements within the ore minerals. For example, the most common ore of copper is chalcopyrite (CuFeS2). The copper needs to be separated from the iron and sulphur to make copper metal and that involves complicated and very energy-intensive processes that are done at smelters or other types of refineries. Because of their cost and the economies of scale, there are far fewer refineries than there are mines.
There are several metal refineries (including smelters) in Canada; some examples are the aluminum refinery in Kitimat, B.C. (which uses ore from overseas); the lead-zinc smelter in Trail, B.C.; the nickel smelter at Thompson, Manitoba; numerous steel smelters in Ontario, along with several other refining operations for nickel, copper, zinc, and uranium; aluminum refineries in Quebec; and a lead smelter in New Brunswick.
18.4 Industrial Minerals
Metals are critical for our technological age, but there are a lot of other not-so-shiny materials that are needed to facilitate our way of life. For everything made out of concrete or asphalt, we need sand and gravel. To make the cement that holds concrete together, we also need limestone. For the glass in our computer screens and for glass-sided buildings, we need silica sand plus sodium oxide (Na2O), sodium carbonate (Na2CO3), and calcium oxide (CaO). Potassium is an essential nutrient for farming in many areas, and for a wide range of applications (e.g., ceramics and many industrial processes), we also need various types of clay.
The best types of aggregate (sand and gravel) resources are those that have been sorted by streams, and in Canada the most abundant and accessible fluvial deposits are associated with glaciation. That doesn’t include till of course, because it has too much silt and clay, but it does include glaciofluvial outwash, which is present in thick deposits in many parts of the country, similar to the one shown in Figure 18.17. In a typical gravel pit, these materials are graded on-site according to size and then used in a wide range of applications from constructing huge concrete dams to filling children’s sandboxes. Sand is also used to make glass, but for most types of glass, it has to be at least 95% quartz (which the sandy layers shown in Figure 18.17 are definitely not), and for high-purity glass and the silicon wafers used for electronics, the source sand has to be over 98% quartz.
![Sand and gravel in an aggregate pit near Nanaimo, BC. [SE]](figures/18-geological-resources/figure-18-15.jpg)
Figure 18.17: Sand and gravel in an aggregate pit near Nanaimo, BC. [SE]
Approximately 80 million tonnes of concrete are used in Canada each year — a little over 2 tonnes per person. The cement used for concrete is made from approximately 80% calcite (CaCO3) and 20% clay. This mixture is heated to 1450°C to produce the required calcium silicate compounds (e.g., Ca2SiO4). The calcite typically comes from limestone quarries like the one on Texada Island, B.C. (Figure 18.18). Limestone is also used as the source material for many other products that require calcium compounds, including steel and glass, pulp and paper, and plaster products for construction.
![Triassic Quatsino Formation limestone being quarried on Texada Island, B.C. [SE]](figures/18-geological-resources/figure-18-16.jpg)
Figure 18.18: Triassic Quatsino Formation limestone being quarried on Texada Island, B.C. [SE]
Sodium is required for a wide range of industrial processes, and the most convenient source is sodium chloride (rock salt), which is mined from evaporite beds in various parts of Canada. The largest salt mine in the world is at Goderich, Ontario, where salt is recovered from the 100 m thick Silurian Salina Formation. The same formation is mined in the Windsor area. Rock salt is also used as a source of sodium and chlorine in the chemical industry to melt ice on roads, as part of the process of softening water, and as a seasoning. Under certain conditions, the mineral sylvite (KCl) accumulates in evaporite beds, and this rock is called potash. This happened across the Canadian prairies during the Devonian, creating the Prairie evaporite formation (Figure 18.18). Potassium is used as a crop fertilizer, and Canada is the world’s leading supplier, with most of that production coming from Saskatchewan.
Another evaporite mineral, gypsum (CaSO4.2H20), is the main component of plasterboard (drywall) that is widely used in the construction industry. One of the main mining areas for gypsum in Canada is in the Milford Station area of Nova Scotia, site of the world’s largest gypsum mine.
Rocks are quarried or mined for many different uses, such as building facades (Figure 18.19), countertops, stone floors, and headstones. In most of these cases, the favoured rock types are granitic rocks, slate, and marble. Quarried rock is also used in some applications where rounded gravel isn’t suitable, such as the ballast (road bed) for railways, where crushed angular rock is needed.
![Slate used as a facing material on a concrete building column in Vancouver [SE]](figures/18-geological-resources/figure-18-17.jpg)
Figure 18.19: Slate used as a facing material on a concrete building column in Vancouver [SE]
Exercise: Sources of Important Lighter Minerals
When we think of the manufacture of consumer products, plastics and the heavy metals (copper, iron, lead, zinc) easily come to mind, but we often forget about some of the lighter metals and non-metals that are important. Consider the following elements and determine their sources. Answers for all of these except magnesium are given above. See if you can figure out a likely mineral source of magnesium.
Silicon:
Calcium:
Sodium:
Potassium:
Magnesium:
18.5 Fossil Fuels
There are numerous types of fossil fuels, but all of them involve the storage of organic matter in sediments or sedimentary rocks. Fossil fuels are rich in carbon and almost all of that carbon ultimately originates from CO2 taken out of the atmosphere during photosynthesis. That process, driven by solar energy, involves reduction (the opposite of oxidation) of the carbon, resulting in it being combined with hydrogen instead of oxygen. The resulting organic matter is made up of complex and varied carbohydrate molecules.
Most organic matter is oxidized back to CO2 relatively quickly (within weeks or years in most cases), but any of it that gets isolated from the oxygen of the atmosphere (for example, deep in the ocean or in a stagnant bog) may last long enough to be buried by sediments and, if so, may be preserved for tens to hundreds of millions of years. Under natural conditions, that means it will be stored until those rocks are eventually exposed at the surface and weathered.
In this section, we’ll discuss the origins and extraction of the important fossils fuels, including coal, oil, and gas.
18.5.1 Coal
Coal was the first fossil fuel to be widely used. As we learned in section 9.4, coal forms where vigorous growth of vegetation in swampy areas leads to an abundance of organic matter that accumulates within stagnant water. The chemical nature of the water- it being acidic and having little to no oxygen- means the organic matter decays very little. If a thick layer of organic matter is accumulated and then buried, the organic matter begins to change as it is compressed and heated. This situation, where the dead organic matter is submerged in oxygen-poor water, must be maintained for centuries to millennia in order for enough material to accumulate to form a thick layer. Over time, as the organic matter is heated and compressed more and more, the carbon within it becomes concentrated, and it can provide more energy when it is burned. Figure 18.20 shows the classification system for different grades of coal. Increasing pressure and temperature means proceeding clockwise through the diagram, starting with lignite (the lowest grade), then bituminous coal, and finally anthracite, the highest grade.
![Coal ranking system used by the United States Geological Survey (USGS). As vegetative organic matter is buried deeper, and experiences higher pressures and temperatures, it progresses clockwise through the diagram, beginning with lignite. Additional heat and pressure result in the coal having a higher concentration of carbon (the vertical axis), and producing more energy (the horizontal axis). [USGS, public domain, https://commons.wikimedia.org/wiki/File:Coal_Rank_USGS.png#mw-jump-to-license]](figures/18-geological-resources/figure-18-18.png)
Figure 18.20: Coal ranking system used by the United States Geological Survey (USGS). As vegetative organic matter is buried deeper, and experiences higher pressures and temperatures, it progresses clockwise through the diagram, beginning with lignite. Additional heat and pressure result in the coal having a higher concentration of carbon (the vertical axis), and producing more energy (the horizontal axis). [USGS, public domain, https://commons.wikimedia.org/wiki/File:Coal_Rank_USGS.png#mw-jump-to-license]
There are significant coal deposits in many parts of Canada, including the Maritimes, Ontario, Saskatchewan, Alberta, and British Columbia. In Alberta and Saskatchewan, much of the coal is used for electricity generation. Coal from the Highvale Mine (Figure 18.21), Canada’s largest, is used to feed the Sundance and Keephills power stations west of Edmonton. Almost all of the coal mined in British Columbia is exported for use in manufacturing steel.
![The Highvale Mine (background) and the Sundance (right) and Keephills (left) generating stations on the southern shore of Wabamun Lake, Alberta [SE]](figures/18-geological-resources/figure-18-19.jpg)
Figure 18.21: The Highvale Mine (background) and the Sundance (right) and Keephills (left) generating stations on the southern shore of Wabamun Lake, Alberta [SE]
18.5.2 Oil and Gas
While almost all coal forms on land from terrestrial vegetation, most oil and gas is derived primarily from marine micro-organisms that accumulate within sea-floor sediments. In areas where marine productivity is high, dead organic matter is delivered to the sea floor fast enough that some of it escapes oxidation. This material accumulates in the muddy sediments, which become buried to significant depth beneath other sediments.
As the depth of burial increases, so does the temperature — due to the geothermal gradient — and gradually the organic matter within the sediments is converted to hydrocarbons (Figure 18.22). The first stage is the biological production (involving anaerobic bacteria) of methane. Most of this escapes back to the surface, but some is trapped in methane hydrates near the sea floor. At depths beyond about 2 km, and at temperatures ranging from 60° to 120°C, the organic matter is converted by chemical processes to oil. This depth and temperature range is known as the oil window. Beyond 120°C most of the organic matter is chemically converted to methane.
![The depth and temperature limits for biogenic gas, oil, and thermogenic gas [SE]](figures/18-geological-resources/figure-18-20.png)
Figure 18.22: The depth and temperature limits for biogenic gas, oil, and thermogenic gas [SE]
The organic matter-bearing rock within which the formation of gas and oil takes place is known to petroleum geologists as the source rock. Both liquid oil and gaseous methane are lighter than water, so as liquids and gases form, they tend to move slowly toward the surface, out of the source rock and into reservoir rocks. Reservoir rocks are typically relatively permeable because that allows migration of the fluids from the source rocks, and also facilitates recovery of the oil or gas. In some cases, the liquids and gases make it all the way to the surface, where they are oxidized, and the carbon is returned to the atmosphere. But in other cases, they are contained by overlying impermeable rocks (e.g., mudrock) in situations where anticlines, faults, stratigraphy changes, and reefs or salt domes create traps (Figure 18.23).
![Migration of oil and gas from source rocks into traps in reservoir rocks [SE]](figures/18-geological-resources/figure-18-21.png)
Figure 18.23: Migration of oil and gas from source rocks into traps in reservoir rocks [SE]
The liquids and gases that are trapped within reservoirs become separated into layers based on their density, with gas rising to the top, oil below it, and water underneath the oil. The proportions of oil and gas depend primarily on the temperature in the source rocks. Some petroleum fields, such as many of those in Alberta, are dominated by oil, while others, notably those in northeastern B.C., are dominated by gas.
![Seismic section through the East Breaks Field in the Gulf of Mexico. The dashed red line marks the approximate boundary between deformed rocks and younger undeformed rocks. The wiggly arrows are interpreted migration paths. The total thickness of this section is approximately 5 km. [SE after http://wiki.aapg.org/File:Sedimentary-basin-analysis_fig4-55.png]](figures/18-geological-resources/figure-18-22.png)
Figure 18.24: Seismic section through the East Breaks Field in the Gulf of Mexico. The dashed red line marks the approximate boundary between deformed rocks and younger undeformed rocks. The wiggly arrows are interpreted migration paths. The total thickness of this section is approximately 5 km. [SE after http://wiki.aapg.org/File:Sedimentary-basin-analysis_fig4-55.png]
In general, petroleum fields are not visible from the surface, and their discovery involves the search for structures in the subsurface that have the potential to form traps. Seismic surveys are the most commonly used tool for early-stage petroleum exploration, as they can reveal important information about the stratigraphy and structural geology of subsurface sedimentary rocks. An example from the Gulf of Mexico south of Texas is shown in Figure 18.24. In this area, a thick evaporite deposit (“salt”) has formed domes because salt is lighter than other sediments and tends to rise slowly toward the surface; this has created traps. The sequence of deformed rocks is capped with a layer of undeformed rock.
Exercise: Interpreting a Seismic Profile
The cross-section shown here is from a ship-borne seismic survey in the Bering Sea off the west coast of Alaska. As a petroleum geologist, it’s your job to pick two separate locations to drill for oil or gas. Which locations would you choose?
[from USGS at: http://walrus.wr.usgs.gov/infobank/programs/html/definition/seis.html]
18.5.2.1 What Is Unconventional Oil and Gas?
The type of oil and gas reservoirs illustrated in Figures 18.23 and 18.24 are described as conventional reserves. Some unconventional types of oil and gas include oil sands, shale gas, and coal-bed methane.
18.5.2.1.1 Oil Sands
Oil sands are important because the reserves in Alberta are so large (the largest single reserve of oil in the world), but they are very controversial from an environmental and social perspective. They are “unconventional” because the oil is exposed near the surface and is highly viscous because of microbial changes that have taken place at the surface. The hydrocarbons that form this reserve originated in deeply buried Paleozoic rocks adjacent to the Rocky Mountains and migrated up and toward the east (Figure 18.25).
The oil sands are controversial primarily because of the environmental cost of their extraction. Since the oil is so viscous, it requires heat to make it sufficiently liquid to process. This energy comes from gas; approximately 25 m3 of gas is used to produce 0.16 m3 (one barrel) of oil. (That’s not quite as bad as it sounds, as the energy equivalent of the required gas is about 20% of the energy embodied in the produced oil.) The other environmental cost of oil sands production is the devastation of vast areas of land where strip-mining is taking place and tailings ponds are constructed, and the unavoidable release of contaminants into the groundwater and rivers of the region.
At present, most oil recovery from oil sands is achieved by mining the sand and processing it on site. Exploitation of oil sand that is not exposed at the surface depends on in situ processes, an example being the injection of steam into the oil-sand layer to reduce the viscosity of the oil so that it can be pumped to the surface.
![Schematic cross-section of northern Alberta showing the source rocks and location of the Athabasca Oil Sands [SE]](figures/18-geological-resources/figure-18-23.png)
Figure 18.25: Schematic cross-section of northern Alberta showing the source rocks and location of the Athabasca Oil Sands [SE]
18.5.2.1.2 Shale Gas
Shale gas is gas that is trapped within rock that is too impermeable for the gas to escape under normal conditions, and it can only be extracted by fracturing the reservoir rock using water and chemicals under extremely high pressure. This procedure is known as hydraulic fracturing or “fracking.” Fracking is controversial because of the volume of water used, and because, in some jurisdictions, the fracking companies are not required to disclose the nature of the chemicals used. Although fracking is typically done at significant depths, there is always the risk that overlying water-supply aquifers could be contaminated (Figure 18.26). Fracking also induces low-level seismicity (earthquakes).
![Depiction of the process of directional drilling and fracking to recover gas from impermeable rocks. The light blue arrows represent the potential for release of fracking chemicals to aquifers. [by SE, after https://en.wikipedia.org/wiki/Hydraulic_fracturing#/media/File:HydroFrac2.svg]](figures/18-geological-resources/figure-18-24.png)
Figure 18.26: Depiction of the process of directional drilling and fracking to recover gas from impermeable rocks. The light blue arrows represent the potential for release of fracking chemicals to aquifers. [by SE, after https://en.wikipedia.org/wiki/Hydraulic_fracturing#/media/File:HydroFrac2.svg]
During the process that converts organic matter to coal, some methane is produced, which is stored within the pores of the coal. When coal is mined, methane is released into the mine where it can become a serious explosion hazard. Modern coal-mining machines have methane detectors on them and actually stop operating if the methane levels are dangerous. It is possible to extract the methane from coal beds without mining the coal; gas recovered this way is known as coal-bed methane.
18.6 Diamonds
Although Canada’s diamond mining industry didn’t get started until 1998, diamonds are currently the sixth most valuable product mined in the country (Figure 18.4), and Canada ranks sixth in the world in diamond production. Diamonds form deep in the mantle (approximately 200 km to 250 km depth) under very specific pressure and temperature conditions, from carbon that is naturally present in mantle rock (not from coal). The diamond-bearing rock is brought to the surface coincidentally via a type of volcanism that is extremely rare (the most recent kimberlite eruption is thought to have taken place 10,000 years ago and prior to that at around 30 Ma). There is more on the volcanology of kimberlites in section 11.3. All of the world’s kimberlite diamond deposits are situated within ancient shield areas (cratons) in Africa, Australia, Russia, South America, and North America.
It has long been known that diamonds could exist within the Canadian Shield, but up until 1991, exploration efforts had been unsuccessful. In 1980 two geologists, Chuck Fipke and Stu Blusson, started searching in the Northwest Territories by sampling glacial sediments looking for some of the minerals that are normally quite abundant within kimberlites: chromium-bearing garnet, chromium-bearing pyroxene, chromite (Cr2O3), and ilmenite (FeTiO3). These distinctive minerals are used for this type of exploration because they are many times more abundant in kimberlite than diamond is. After more than a decade of exploration, Fipke and Blusson finally focused their search on an area 250 km northeast of Yellowknife, and, in 1991, they announced the discovery of a diamond-bearing kimberlite body at Lac de Gras. That discovery is now the Diavik Mine, and there is another diamond mine — Ekati — 25 km to the northwest (Figure 18.27). There are two separate mines at Diavik accessing three different kimberlite bodies, and there are five at Ekati. See Figure 11.22 for a close-up view of the Ekati Mine. There are six operating diamond mines in Canada, four in the Northwest Territories (including Diavik and Ekati), and one each in Nunavut and Ontario.
![Diamond mines in the Lac de Gras region, Nunavut. The twin pits of the Diavik Mine are visible in the lower right on an island within Lac de Gras. The five pits of the Ekati mine are also visible, on the left and the upper right. The two main mine centres are 25 km apart. [http://earthobservatory.nasa.gov/IOTD/view.php?id=84085&src=eoa-iotd]](figures/18-geological-resources/figure-18-25.jpg)
Figure 18.27: Diamond mines in the Lac de Gras region, Nunavut. The twin pits of the Diavik Mine are visible in the lower right on an island within Lac de Gras. The five pits of the Ekati mine are also visible, on the left and the upper right. The two main mine centres are 25 km apart. [http://earthobservatory.nasa.gov/IOTD/view.php?id=84085&src=eoa-iotd]
18.7 Summary
The main topics of this chapter can be summarized as follows:
18.7.1 If You Can’t Grow It, You Have To Mine It
Geological resources are critical to our way of life and important to the Canadian economy. Gold, iron, copper, nickel, and potash are Canada’s most valuable mined commodities.
18.7.2 Metal Deposits
The proportions of metals in mineral deposits are typically several thousand times higher than those in average rocks, and special processes are required to extract the valuable content. Some deposits form through processes within a magma chamber, others during volcanism or adjacent to a stock, and some are related to sedimentary processes. Mining involves both surface and underground methods, but in either case, rock is brought to surface that can react with water and oxygen to produce acid rock drainage and metal contamination.
18.7.3 Industrial Materials
Non-metallic materials are very important to infrastructure and agriculture. Some of the major industrial minerals include sand and gravel, limestone for cement and agriculture, salt for a range of applications, potash fertilizer, and decorative stone.
18.7.4 Fossil Fuels
The main fossil fuels are coal, oil, and gas. Coal forms on land in wet environments where organic matter can remain submerged and isolated from oxygen for millennia before it is buried by more sediments. The depth of that burial influences the grade of coal produced. Oil and gas originate from organisms living in marine environments, and again, fairly rapid burial is required to preserve the organic matter on the sea floor. At moderate burial depth (2 km to 4 km), oil is produced, and at greater depth, gas is produced. Both oil and gas migrate toward the surface and can be trapped beneath impermeable rock layers in structural features, such as anticlines or faults. Some unconventional fossil fuel resources include oil sands, shale gas, and coal-bed methane.
18.7.5 Diamonds
Diamonds originate in the mantle and are only brought to the surface by the very rare eruption of kimberlitic volcanoes. The relatively recent discovery of diamonds in Canada was based on the exhaustive search for diamond indicator minerals in glacial sediments. There are now six diamond mines in Canada.
18.8 Chapter Review Questions
- What are some of Earth’s resources that are needed to make a compact fluorescent light bulb? The image shows the contents of the ballast.
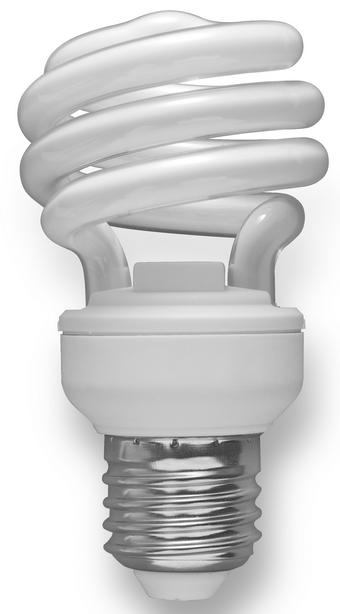 https://upload.wikimedia.org/wikipedia/commons/3/31/06_Spiral_CFL_Bulb_2010-03-08_%28white_back%29.jpg](https://openpress.usask.ca/app/uploads/sites/29/2017/05/bulb.png)
Figure 18.28: https://upload.wikimedia.org/wikipedia/commons/3/31/06_Spiral_CFL_Bulb_2010-03-08_%28white_back%29.jpg
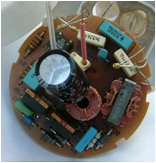 https://en.wikipedia.org/wiki/ Compact_fluorescent_lamp<br>#/ media/File:Elektronstarterp.jpg](https://openpress.usask.ca/app/uploads/sites/29/2017/05/Compact_fluorescent_lamp.png)
Figure 18.29: https://en.wikipedia.org/wiki/ Compact_fluorescent_lamp
#/ media/File:Elektronstarterp.jpg
Explain why nickel deposits are associated only with mafic magma and not with intermediate or felsic magma?
What is the composition of the black smoke in a black smoker, and how does that relate to a volcanogenic massive sulphide deposit?
How might an epigenetic gold deposit be related to a porphyry deposit?
Oxidation and reduction processes are important to both banded iron formation deposits and unconformity-type uranium deposits. Explain the role in each case.
A typical kimberlite in northern Canada may look something like the diagram shown. In this case, the diameter at the surface is around 500 m, and the total depth is about 2,500 m. Bearing in mind that an open pit cannot typically be any deeper than it is wide, what mining method(s) might be most applicable to a deposit of this type?
What mineral is typically responsible for acid rock drainage around mine sites, and why is this mineral so common in this setting?
Explain why glaciofluvial gravel is more suitable than till as a source for aggregate.
The raw material for making cement is lime (CaO), and this is typically produced by heating limestone (mostly CaCO3) to about 1,000°C. Why is this an environmental issue?
Name some important industrial minerals that form in an evaporite setting.
If organic matter accumulates at an average rate of 1 mm per year, and if 10 m of organic matter is required to make 1 m of coal, how long must a swampy environment remain stable and wet in order to form a 1.5 m coal seam?
What are the ideal characteristics of petroleum source rocks and petroleum reservoir rocks?
How deep must the source rocks be buried to produce oil?
Why is shale gas an unconventional reserve, and how is it recovered? What are some of the environmental issues associated with that process?
If you were sampling glacial deposits to search for kimberlites, why would you be advised to look for kimberlite indicator minerals rather than diamonds?
18.9 Answers to Chapter Review Questions
Some of the components of a compact fluorescent lightbulb (and the resources used to make one) are as follows:
- Steel (iron, carbon from coal plus some manganese, nickel, chromium, molybdenum)
- Plastic housing (petroleum)
- Glass coil (silica from sand, plus minor amounts of sodium, calcium, and magnesium)
- Copper conductors, lead solder, and basal contact
- Silica (sand), plastics (petroleum), ceramics (clay), aluminum, gold, copper, etc. in the electronics
- Mercury inside the tube (less than 5 mg)
Nickel deposits form within mafic and ultramafic igneous bodies because the original magma have relatively high nickel levels to begin with, while intermediate or felsic magma have low levels.
The “smoke” in a black smoker is composed of tiny crystals of sulphide minerals. If those include significant quantities of ore minerals like chalcopyrite (CuFeS2), sphalerite (ZnS), and galena (PbS), a VMS deposit could form during this process.
A porphyry deposit is situated in the rock around an igneous pluton that has intruded to a relatively high level in the crust (and hence is porphyritic), and they form at least in part from fluids released by the magma. Epigenetic gold deposits may be formed from the same or similar fluids, but are situated at a greater distance from the pluton/
Ferrous iron (Fe2+) is soluble in water with a low oxidation potential, and gets converted to insoluble ferric iron (Fe3+) when the water becomes oxidized. The opposite situation happens with uranium. Uranyl uranium (U6+) is soluble under oxidizing conditions, but when the water in which it is dissolved encounters reducing conditions the uranium is converted to the insoluble uranous ion (U4+).
It is common for the upper part of a kimberlite to be mined using an open pit (in this case around 500 m wide and up to 500 m deep), and for the lower part to be mined underground.
Pyrite (FeS2) is typically responsible for acid rock drainage around mine sites, and it is very common for pyrite to form within the rock at the same time that other metal sulphides (e.g., chalcopyrite) are forming.
Glaciofluvial gravels are typically relatively well sorted, and may include clasts ranging in size from coarse sand to pebbles. Till, on the other hand, tends to be poorly sorted and may have clasts ranging from clay to boulders. More processing would be needed to separate the required size ranges, and because till tends to be relatively hard and strong, this would require a lot of effort.
During the manufacture of CaO limestone is heated and CO2 is released to the atmosphere, adding to the greenhouse effect. The energy required for this process typically comes from fossil fuels (e.g., natural gas) and the combustion also releases CO2.
Some important evaporite minerals include halite (NaCl), sylvite (KCl), and gypsum (CaSO4.2H2O).
The 15 m of organic matter required to make 1.5 m of coal, is equivalent to 15,000 mm, and if the organic matter accumulates at 1 mm/y that would require 15,000 years. That organic matter would have to remain submerged in oxygen-poor water for at least that length of time.
Petroleum source rocks must have a significant component of organic matter, and then need to be buried to at least 2,500 m depth so that the organic matter can be converted to oil or gas. Reservoir rocks must be both porous and permeable, so that the petroleum liquids can be extracted, and should also take the form of a trap (e.g., an anticline) and capped with impermeable rock.
The optimum depth for the generation of oil from buried organic matter is 2,500 to 3,500 m.
Shale gas is an unconventional reserve because shale is not permeable enough to allow the gas to be extracted. The rock has to be fractured (fracked) to allow recovery. Fracking involves the use of vast amounts of water, and there is the potential that the fracking fluids can contaminate freshwater aquifers.
Kimberlite indicator minerals are much more abundant than diamonds within kimberlites, and so they can typically be detected further away from the kimberlite source, and over a much wider area.