Chapter 11 Volcanism
Adapted from Physical Geology, First University of Saskatchewan Edition (Karla Panchuk) and Physical Geology (Steven Earle)
 Click the image for more attributions._](figures/11-volcanism/figure-11-1.png)
Figure 11.1: Mt. Garibaldi (in the background), near Squamish BC, is one of Canada’s most recently active volcanoes, last erupted approximately 10,000 years ago. It is also one of the tallest, at 2,678 m in height. Source: Karla Panchuk (2017) CC BY-SA 4.0. Photograph: Michael Scheltgen (2006) CC BY 2.0 view source Click the image for more attributions.
Learning Objectives
After reading this chapter and answering the Questions For Review at the end, you should be able to:
- Explain what a volcano is.
- Describe the different kinds of materials produced by volcanoes.
- Describe the structures of shield volcanoes, composite volcanoes, and cinder cones.
- Explain how the style of a volcanic eruption is related to magma composition.
- Describe the role of plate tectonics in volcanism and magma formation.
- Summarize the hazards that volcanic eruptions pose to people and infrastructure.
- Describe how volcanoes are monitored, and the signals that indicate a volcano could be ready to erupt.
- Provide an overview of Canadian volcanic activity.
11.0.1 Why Study Volcanoes?
Volcanoes are awe-inspiring natural events. They have instilled fear and fascination with their red-hot lava flows, and cataclysmic explosions. In his painting The Eruption of Vesuvius (Figure 11.2), Pierre-Jacques Volaire captured the stunning spectacle of the eruption on Mt. Vesuvius on 14 May 1771. He also captured some stunningly casual spectating being done by tourists and their dog (lower left).
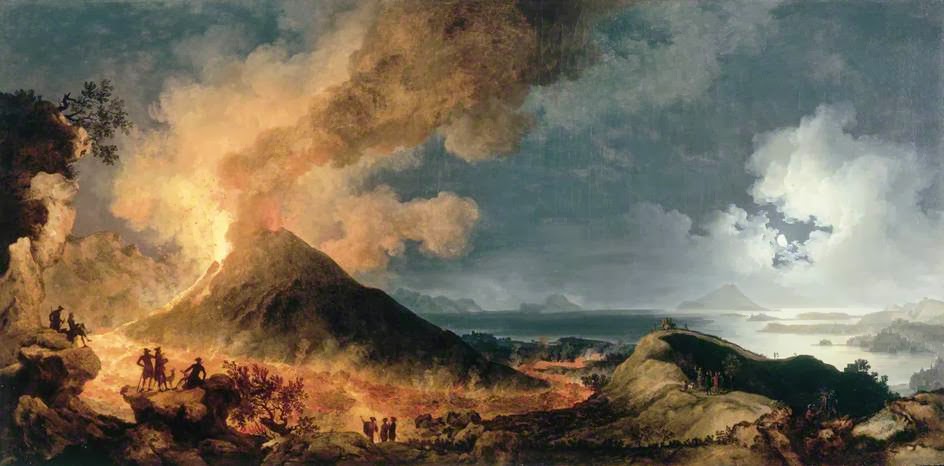
Figure 11.2: The Eruption of Vesuvius, by Pierre-Jacques Volaire (1771). Public Domain.
As Volaire’s painting suggests, curiosity alone would be enough to make people want to learn why volcanoes happen and how they work. However, there are other reasons why we should know more about volcanoes. One reason is that studying volcanoes helps us understand the evolution of the Earth system- not just Earth’s geological features, but past changes in climate, and even the causes of mass extinctions. Another reason is the critical need to study the hazards posed by volcanoes to people and infrastructure. Over the past few decades, volcanologists have made great strides in their ability to forecast volcanic eruptions and predict the consequences, saving thousands of lives.
11.1 What Is A Volcano?
11.1.1 Volcanoes Are Where Magma Emerges
A __volcano __is a location where molten rock flows out, or erupts, onto Earth’s surface as lava. Volcanic eruptions can happen on land or underwater. Some volcanic eruptions flow from mountains (such as Mount Garibaldi in Figure 11.1), but others do not. Fissure eruptions (Figure 11.3) are volcanic eruptions flowing from long cracks in the Earth.
. Click the image for more attributions._](figures/11-volcanism/figure-11-3.png)
Figure 11.3: Kamoamoa fissure eruption on the flanks of the Hawai’ian Kīlauea Volcano in March of 2011. Source: Karla Panchuk (2017) CC BY-SA 4.0. Photograph: U. S. Geological Survey (2011) Public Domain view source. Click the image for more attributions.
11.1.2 Volcano Anatomy
The main parts of a volcano are shown in Figure 11.4 When volcanoes erupt, magma moves upward from a __magma chamber __and into a __vent __or conduit. It flows out from a crater at the top, or sometimes emerges at a secondary site on the side of the volcano resulting in a flank eruption. Erupted materials accumulate around the vent forming a volcanic mountain. The accumulated material might consist of layers of solidified lava, called lava flows, but it might also include fragments of various sizes that have been thrown from the volcano.
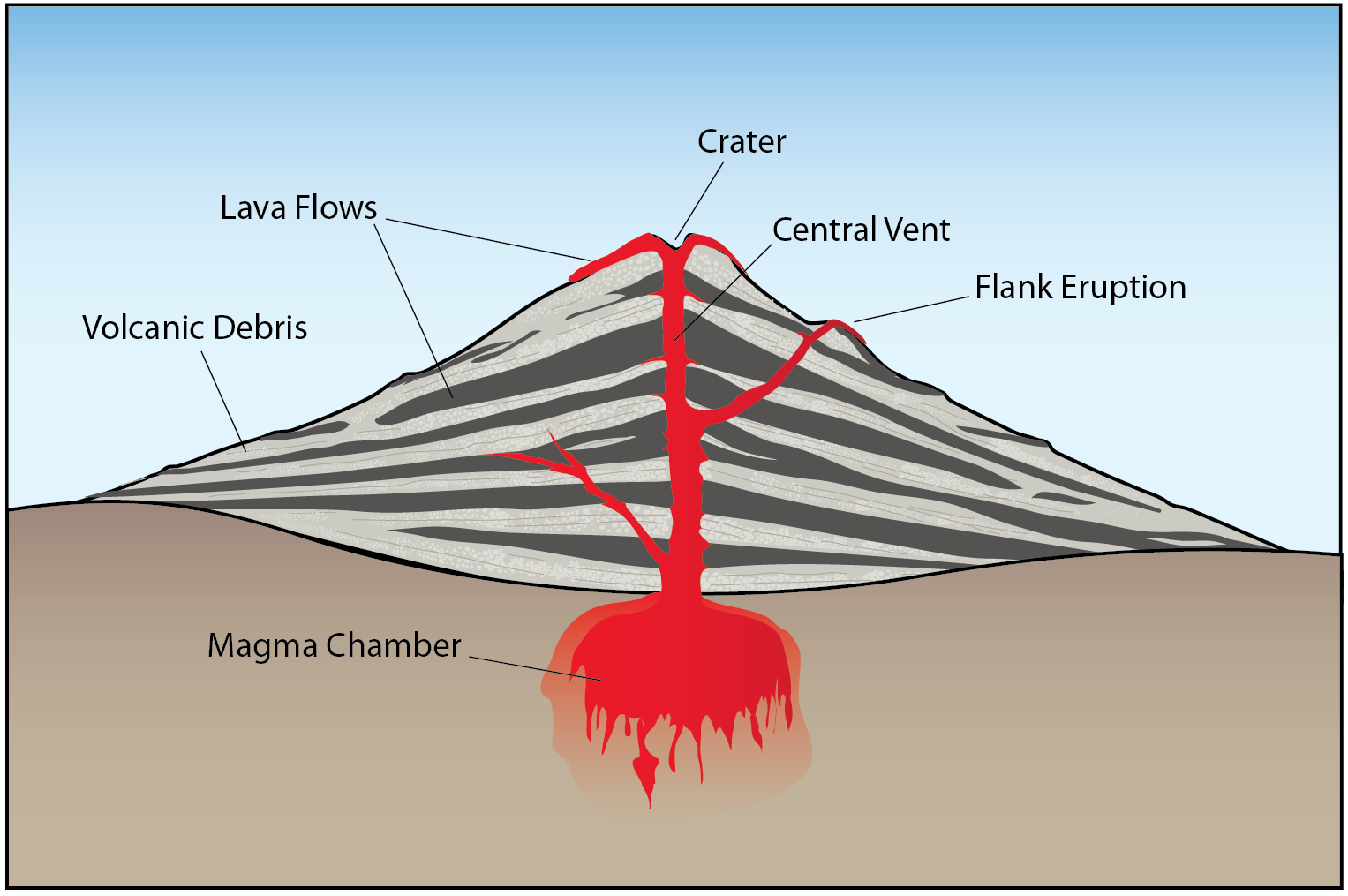
Figure 11.4: The parts of a volcano (not to scale). Source: Karla Panchuk (2017) CC BY 4.0
11.1.2.1 Crater or Caldera?
A crater is the basin above a volcano’s vent. Craters have diameters on the scale of 10s to 100s of metres. A __caldera __is a bowl-shaped structure that resembles a crater, but it is much larger (km in scale) and forms when a volcano collapses in on itself. The process is illustrated in Figure 11.5, going from left to right. It begins when an eruption occurs, and the magma chamber beneath the volcano is drained. If a significant part of a volcano’s mass is supported by magma within the chamber, then depleting the magma also reduces the support for the volcano. The loss of support causes part of the volcano to collapse into the void in the magma chamber, leaving behind a broad basin rimmed by the remnants of the volcano. Over time, the basin can fill with water. If there is still activity within the magma chamber, magma may force its way upward again, causing the floor of the caldera to be lifted, or erupting to form a new volcano within the caldera.
_](figures/11-volcanism/figure-11-5.png)
Figure 11.5: Formation of a caldera. Calderas are the result of a volcano collapsing into a drained magma chamber. Source: Karla Panchuk (2017) CC BY 4.0. Modified after U. S. Geological Survey (2002) Public Domain view source
The island of Santorini (Figure 11.6) is an example of a caldera. The island itself is the rim of the caldera, and the bay is the flooded basin. The two small islands in the middle of the bay formed from magma refilling the chamber that feeds the volcano, as in the far right of Figure 11.5. The caldera formed after an enormous eruption between 1627 and 1600 BCE19. The eruption is thought to have contributed to the downfall of the Minoan civilization, and some speculate that it might also be the source of the myth of Atlantis, a story about a lost continent that sank beneath the sea after a natural disaster.
; Caldera photograph: Klearchos Kapoutsis (2010) CC BY 2.0 [view source](https://flic.kr/p/7PKuhk) Click the image for more attributions._](figures/11-volcanism/figure-11-6.png)
Figure 11.6: The Greek Island of Santorini. Left: Aerial view of the island forming a ring around a flooded caldera. Right: A view from the rim of the caldera. The other side of the rim is visible in the distance. Source: Karla Panchuk (2017) CC BY-SA 4.0. Satellite image: NASA/GSFC/MITI/ERSDAC/JAROS, and U.S./Japan ASTER Science Team (2000) Public Domain view source; Caldera photograph: Klearchos Kapoutsis (2010) CC BY 2.0 view source Click the image for more attributions.
11.2 Materials Produced by Volcanic Eruptions
Volcanic eruptions produce three types of materials: gas, lava, and fragmented debris called tephra.
11.2.1 Volcanic Gas
Magma contains gas. At high pressures, the gases are dissolved within magma. However, if the pressure decreases, the gas comes out of solution, forming bubbles. This process is analogous to what happens when a pop bottle is opened. Pop is bottled under pressure, forcing carbon dioxide gas to dissolve into the fluid. As a result, a bottle of pop that you find on the supermarket shelf will have few to no bubbles. If you open the bottle, you decrease the pressure within it. The pop will begin to fizz as carbon dioxide gas comes out of solution and forms bubbles.
The main component of volcanic gas emissions is water vapour, followed by carbon dioxide (CO2), sulphur dioxide (SO2), and hydrogen sulphide (H2S).
Volcanoes release gases when erupt, and through openings called fumaroles (Figure 11.7). They can also release gas into soil and groundwater.
<br>_](figures/11-volcanism/figure-11-7.jpeg)
Figure 11.7: A fumarole at Puʻu ʻŌʻō Crater, Hawaii. The yellow crust along the margin of the fumarole is made of sulphur crystals. The crystals form when sulphur vapour cools as it is released from the fumarole. Source: U. S. Geological Survey (2016) Public Domain View source
11.2.2 Lava
The ease with which lava flows and the structures it forms depend on how much silica and gas the lava contains. The more silica, the more polymerization (formation of long molecules) occurs, stiffening the lava. The stiffness of lava is described in terms of viscosity- lava that flows easily has low viscosity, and lava that is sticky and stiff has high viscosity.
In general, high-silica lava contains more gas than low-silica lava. When the gas forms into bubbles, viscosity increases further. Consider the pop analogy again. If you were to shake the bottle vigorously and then open it, the pop would come gushing out in a thick, frothy flow. In contrast, if you took care to not shake the bottle before opening it, you could pour out a thin stream of fluid.
11.2.2.1 Chemical Composition Affects the Thickness and Shape of Lava Flows
The thickness and shape of a lava flow depends on its viscosity. The greater the viscosity, the thicker the flow, and the shorter the distance it travels before solidifying. Highly viscous lava might not flow very far at all, and simply accumulate as a bulge, called a lava dome, in a volcano’s crater. Figure 11.8 shows a dome formed from rhyolitic lava in the crater of Mt. St. Helens.
_](figures/11-volcanism/figure-11-8.jpeg)
Figure 11.8: Lava dome in the crater of Mt. St. Helens. Source: Terry Feuerborn (2011) CC BY-NC 2.0 view source
Less viscous rhyolitic lava can travel further, as with the thick flow in Figure 11.9 (right). The left of Figure 11.9 shows thin streams of freely-flowing, low-silica, low-viscosity basaltic lava.
; Right- Michelle Combs, U. S. Geological Survey (2015) Public Domain [view source](https://www.flickr.com/photos/usgeologicalsurvey/22432739869/in/album-72157637377510893/)_](figures/11-volcanism/figure-11-9.png)
Figure 11.9: Lava flows. Left: A geologist collects a sample from a basaltic lava flow in Hawaii. Right: an andesitic lava flow from Kanaga Volcano in the Aleutian Islands. Source: Left- U. S. Geological Survey (2014) Public Domain view source; Right- Michelle Combs, U. S. Geological Survey (2015) Public Domain view source
Low-viscosity basaltic lava flows may travel extended distances if they move through conduits called lava tubes. These are tunnels within older solidified lava flows. Figure 11.10 (top) shows a view into a lava tube through a hole in the overlying rock, called a skylight. Figure 11.10 (bottom) shows the interior of a lava tube, with a person for scale. Lava tubes form naturally and readily because flowing mafic lava preferentially cools near its margins, forming solid lava levées that eventually close over the top of the flow. Lava within tubes can flow for 10s of km because the tubes insulate the lava from the atmosphere and slow the rate at which the lava cools. The Hawai’ian volcanoes are riddled with thousands of old, drained lava tubes, some as long as 50 km.
 Bottom: Thomas Shahan (2013) CC BY-NC 2.0 [view source](https://flic.kr/p/s3RDMf)<br>_](figures/11-volcanism/figure-11-10.png)
Figure 11.10: Lava tubes. Top: An opening in the roof of a lava tube (called a skylight) permitting a view of lava flowing through the tube (Puʻu ʻŌʻō crater, Kīlauea). The opening is approximately 6 m across. Bottom: Inside a lava tube that channelled lava away from Mt. St. Helens in an eruption 1,895 years ago. Sources: Top: U. S. Geological Survey (2016) Public Domain. view source Bottom: Thomas Shahan (2013) CC BY-NC 2.0 view source
11.2.2.2 Lava Structures
11.2.2.2.1 Pahoehoe
Lava flowing on the surface can take on different shapes as it cools. Basaltic lava with an unfragmented surface, like that in Figure 11.9 (right), is called pahoehoe. (pronounced pa-hoy-hoy). Pahoehoe can be smooth and billowy. It can also develop a wrinkled texture, called ropy lava, as shown in Figure 11.11. Ropy lava forms when the outermost layer of the lava cools and develops a skin (visible as a dark layer in Figure 11.11, left), but the skin is still hot and thin enough to be flexible. The skin is stiffer than the lava beneath it, and is dragged by flowing lava and folded up into wrinkles. Figure 11.11 (right) is a close-up view after a cut has been made to show the internal structure of a wrinkled lava flow. Notice the many holes, or vesicles, within the lava, formed when the lava solidified around gas bubbles.
; Right: Fiddledydee (2011) CC BY-NC 2.0 [view source](https://flic.kr/p/9RqSoY)._](figures/11-volcanism/figure-11-11.png)
Figure 11.11: Ropy lava from Hawaii. Left: Ropy texture forming as a thin surface layer of lava cools and is wrinkled by the motion of lava flowing beneath it. Right: Cross-section view of ropy lava. Sources: Left: Z. T. Jackson (2005) CC BY NC-ND 2.0 view source; Right: Fiddledydee (2011) CC BY-NC 2.0 view source.
11.2.2.2.2 A’a and Blocky Lava
If the outer layer of the lava flow cannot accommodate the motion of lava beneath by deforming smoothly, the outer layer will break into fragments as lava moves beneath it. This could happen if the lava flow develops a thicker, more brittle outer layer, or if it moves faster. The result is a sharp and splintery rubble-like lava flow called a’a (pronounced like “lava” but without the l and v). Figure 11.12 (left) shows a close-up view of the advancing front of an a’a lava flow (the flow is moving toward the viewer). Figure 11.12 (right) shows an a’a lava flow viewed from the side. Compare the texture of the a’a flow with the texture of the lighter-grey pahoehoe lava in the foreground of the picture.
; Pacaya aa: Greg Willis (2008) CC BY-SA 2.0 (labels added) [view source](https://commons.wikimedia.org/wiki/File:Pacaya_Volcano_-_Guatemala_(4251539562).jpg).](figures/11-volcanism/figure-11-12.png)
Figure 11.12: Aa lava flows. Left: Close-up view of a’a forming during an eruption of Pacaya Volcano in Guatemala. Field of view approximately 1 m across. Right: Rubbly reddish-brown a’a lava flow viewed from Chain of Craters Road, Hawai’i Volcanoes National Park. Pahoehoe is visible in lighter grey in the foreground. Sources: Photo of Hawaiian aa and pahoehoe: Roy Luck (2009) CC BY 2.0 view source; Pacaya aa: Greg Willis (2008) CC BY-SA 2.0 (labels added) view source.
Higher viscosity andesitic lava flows also develop a fragmented surface, called blocky lava. This is visible in the toe of the andesitic lava flow from Figure 11.9 (right). The difference between a’a and the andesitic blocky lava is that the blocky lava has fragments with smoother surfaces and fewer vesicles.
11.2.2.2.3 Lava Pillows
When lava flows into water, the outside of the lava cools quickly, making a tube (Figure 11.13 (top left)). Blobs of lava develop at the end of the tube (Figure 11.13 (top right)), forming pillows. The bottom left of Figure 10.13 shows pillows covering the sea floor, and the bottom right shows the distinctive rounded shape of pillows in outcrop. Because pillows always form underwater, finding them in the rock record gives us information that the environment was underwater.
; Top right- NSF and NOAA (2010) CC BY 2.0 [view source](https://flic.kr/p/93kj7x); Bottom left- NOAA Okeanos Explorer Program, Galápagos Rift Expedition 2011 (2011) CC BY 2.0 [view source](https://flic.kr/p/fUzHjD); Bottom right- James St. John (2015) CC BY 2.0 [view source](https://www.flickr.com/photos/jsjgeology/20831596894/in/photolist-xJPnZG-yGzEkB-xRj8DT-yKZWEE-ypdQSG-xRb6LS-yMdGzQ-ypfznY-yFRoTn-xRhtFG-xJPfvd-ypjTgg-yMdDNC-yDwbyJ-yDw3Wu-yNcwpZ-yNcLeT-yKTeKf-yvzLio-yESdbm-xRocM4-HCZyD2-xRiTPD-yNczMR-JEP1z9-yKZQo1-yvzxY3-yvN9BX-yMhBwA-93kj66-K5b5va-qMVzsN-yETq1w-rJP8eL-yGzXie-qdJd4B-MiF3Yi-MWnmFN-LQw7ks-L5GHUt-M8iUGh-yKZE5A-yvFJbK-ypeH93-ypefhf-fQtYNA-dXbbnp-dX9Gux-8jk155-7Xd75s)._](figures/11-volcanism/figure-11-13.png)
Figure 11.13: Pillow lavas. Top left: A tube of lava extruding underwater. Hot lava can be seen through cracks in the wall of the tube. The image is approximately 1 m across. (Pacific Ocean, near Fiji). Top right: The rounded end of a tube with cracks showing the lava within. (Pacific Ocean, near Fiji). Bottom left: sea floor near the Galápagos Islands covered with pillow lavas. Bottom right: A boulder made of 2.7 billion year old pillows derived from the Ely Greenstone in north-eastern Minnesota. Sources: Top left- NSF and NOAA (2010) CC BY 2.0 view source; Top right- NSF and NOAA (2010) CC BY 2.0 view source; Bottom left- NOAA Okeanos Explorer Program, Galápagos Rift Expedition 2011 (2011) CC BY 2.0 view source; Bottom right- James St. John (2015) CC BY 2.0 view source.
11.2.2.2.4 Columnar Joints
When lava flows cool and solidify, they shrink. Long vertical cracks, or joints, form within the brittle rock to allow for the shrinkage. Viewed from above, the joints form polygons with 5, 6, or 7- sides, and angles of approximately 120º between sides (Figure 11.14).
_](figures/11-volcanism/figure-11-14.jpg)
Figure 11.14: Columnar joints viewed from above, Giant’s Causeway, Northern Ireland. Source: Meg Stewart (2012) CC BY-SA 2.0 view source
Figure 11.15 shows a side view of columnar joints in a basaltic lava flow in Iceland.
](figures/11-volcanism/figure-11-15.jpg)
Figure 11.15: Columnar joints in a basaltic lava flow, Svartifoss (Black Fall) Vatnajökull National Park, Iceland. Source: Ron Kroetz (2015) CC BY-ND 2.0. view source
11.2.3 Pyroclastic Materials
The pop bottle analogy illustrates another key point about gas bubbles in fluid, which is that the bubbles can propel fluid. In the same way that shaking a pop bottle to make more bubbles will cause pop to gush out when the bottle is opened, gas bubbles can violently propel lava and other materials from a volcano, creating an explosive eruption.
Collectively, loose material thrown from a volcano is referred to as tephra. Individual fragments are referred to in general terms as pyroclasts, so sometimes tephra is also referred to as__ pyroclastic debris__. Pyroclasts are classified according to size.
11.2.3.1 Volcanic Ash
Particles less than 2 mm in diameter are called volcanic ash. Volcanic ash consists of small mineral grains and glass. Figure 11.16 shows volcanic ash on three scales: in the upper left is ash from the 2010 eruption of Eyjafjallajökull in Iceland. The image was taken with a scanning electron microscope at approximately 1000 times magnification. In the upper right is ash from the 1980 eruption of Mt. St. Helens, collected in Yakima, Washington, about 137 km northeast of Mt. St. Helens. Individual particles are under 1 mm in size. Figure 11.16 (bottom) shows a village near Mt. Merapi in Indonesia dusted in ash after an eruption 2010.
 Upper right: James St. John (2014) CC BY 2.0 (scale added) [view source](https://flic.kr/p/oUPZxZ) Bottom: AusAID/Jeong Park (2010) CC BY 2.0. [view source](https://flic.kr/p/hfkWdo) _](figures/11-volcanism/figure-11-16.png)
Figure 11.16: Volcanic ash. Upper left: Ash from 2010 eruption of Eyjafjallajökull in Iceland, magnified approximately 1000x. Upper right- Ash from the 1980 eruption of Mt. St. Helens, collected at Yakima, Washington. Bottom: Indonesian village after the eruption of Mt. Merapi in 2010. Sources: Upper left: Birgit Hartinger, AEC (2010) CC BY-NC-ND 2.0. view source Upper right: James St. John (2014) CC BY 2.0 (scale added) view source Bottom: AusAID/Jeong Park (2010) CC BY 2.0. view source
11.2.3.2 Lapilli
Fragments with dimensions between 2 mm and 64 mm are classified as lapilli. Figure 11.17 (upper left) shows lapilli at the ancient city of Pompeii, which was buried when Mt. Vesuvius erupted in 79 C.E. Figure 11.17 (lower left) is a form of lapilli called Pele’s tears, named after the Hawai’ian diety Pele. Pele’s tears form when droplets of lava cool quickly as they are flung through the air. Rapidly moving through the air may draw the Pele’s tears out into long threads called Pele’s hair (Figure 11.17, right). The dark masses in Figure 11.17 (right) within the Pele’s hair are Pele’s tears.
; Lower left: James St. John (2014) CC BY 2.0 (scale added) [view source](https://flic.kr/p/oBgw9G); Right: James St. John (2009) CC BY 2.0 (scale added) [view source](https://flic.kr/p/oRJoBA)._](figures/11-volcanism/figure-11-17.png)
Figure 11.17: Lapilli are pyroclasts ranging between 2 mm and 64 mm in size. Upper left: lapilli from the site of the ancient city of Pompeii. Lower left: Pele’s tears, a type of lapilli that forms when droplets of lava fly through the air. Right: Pele’s hair, which form when Pele’s tears are drawn out into thin threads as they fly. Sources: Upper left: Pauline (2009) CC BY-NC-ND 2.0 view source; Lower left: James St. John (2014) CC BY 2.0 (scale added) view source; Right: James St. John (2009) CC BY 2.0 (scale added) view source.
11.2.3.3 Blocks and Bombs
Fragments larger than 64 mm are classified as blocks or bombs, depending on their origin. Blocks are solid fragments of the volcano that form when an explosive eruption shatters the pre-existing rocks. Figure 11.18 shows one of many blocks from an explosive eruption at the Halema‘uma‘u crater at Kīlauea Volcano in May of 1924. The block has a mass of approximately 7 tonnes and landed 1 km from the crater.
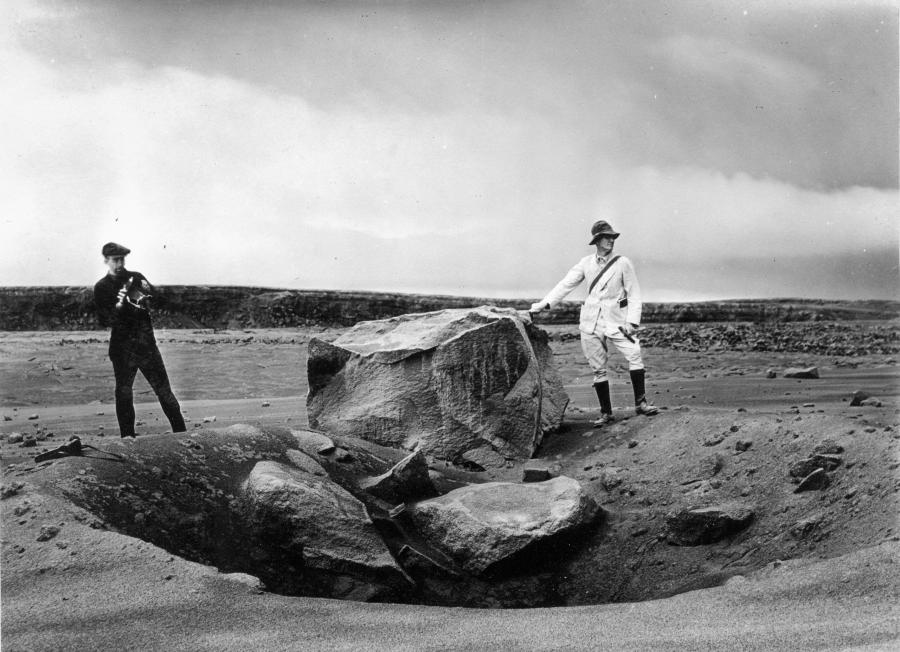_](figures/11-volcanism/figure-11-18.jpg)
Figure 11.18: Volcanic block weighing approximately 7 tonnes thrown 1 km from the Halema‘uma‘u crater at Kīlauea Volcano on May 18, 1924. Source: U. S. Geological Survey (1924) Public Domain view source
Bombs form when lava is thrown from the volcano and cools as it travels through the air. Traveling through the air may cause the lava to take on a streamlined shape, as with the example in Figure 11.19.
_](figures/11-volcanism/figure-11-19.png)
Figure 11.19: Volcanic bomb with a streamlined shape. Source: James St. John (2016) CC BY 2.0 (scale added) view source
11.2.3.4 Effects of Gas on Lapilli and Bombs
The presence of gas in erupting lava can cause lapilli and bombs to take on distinctive forms as the lava freezes around the gas bubbles, giving the rocks a vesicular (hole-filled) texture. Pumice (Figure 11.20) forms from gas-filled felsic lava. Figure 11.20 (right), shows a magnified view of the sample on the left. The dark patches in the photograph are mineral crystals that formed in the magma chamber before the lava erupted. Pumice floats on water because some of the holes are completely enclosed, and air-filled.
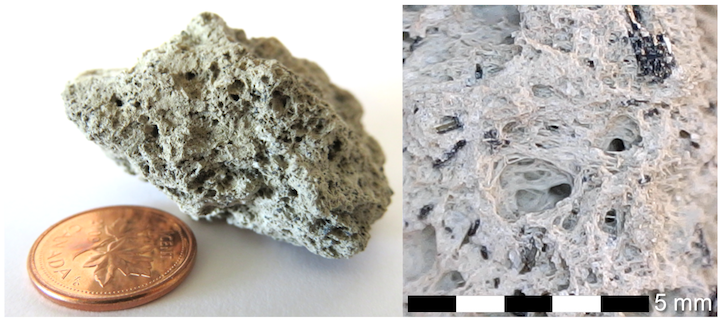
Figure 11.20: Lapilli-sized pumice collected from the shores of Lake Atitlán in Guatemala by H. Herrmann. The lake is a flooded caldera, and is surrounded by active volcanoes. Right: Magnified view showing vesicular structure and amphibole crystals (dark patches). Source: Karla Panchuk (2017) CC BY 4.0
The mafic counterpart to pumice is scoria (Figure 11.21, left). Mafic lava can also form reticulite (Figure 11.21, right), a rare and fragile rock in which the walls surrounding the bubbles have all burst, leaving behind a delicate network of glass.
; Right- James St. John (2014) CC BY 4.0 (scale added) [view source](https://flic.kr/p/oBgu7W)._](figures/11-volcanism/figure-11-21.png)
Figure 11.21: Mafic lapilli with vesicular textures. Left: Scoria from Mount Fuji, Japan. Scoria is the denser mafic counterpart to pumice. Right: Reticulite from Kīlauea Volcano. Reticulite is a delicate network of volcanic glass that forms when the walls separating gas bubbles pop. Sources: Left- James St. John (2014) CC BY 2.0 (scale added) view source; Right- James St. John (2014) CC BY 4.0 (scale added) view source.
11.3 Types of Volcanoes
The products of volcanism that build volcanoes and leave lasting marks on the landscape include lava flows that vary in viscosity and gas content, and tephra ranging in size from less than a mm to blocks with masses of many tonnes. Individual volcanoes vary in the volcanic materials they produce, and this affects the size, shape, and structure of the volcano.
There are three types of volcanoes: cinder cones (also called spatter cones), composite volcanoes (also called stratovolcanoes), and shield volcanoes. Figure 11.22 illustrates the size and shape differences amongst these volcanoes.
Shield volcanoes, which get their name from their broad rounded shape, are the largest. Figure 11.22 shows the largest of all shield volcanoes- in fact, the largest of all volcanoes on Earth- Mauna Loa, which makes up a substantial part of the Island of Hawai‘i and has a diameter of nearly 200 km. The summit of Mauna Loa is presently 4,169 m above sea level, but this represents only a small part of the volcano. It rises up from the ocean floor at a depth of approximately 5,000 m. Furthermore, the great mass of the volcano has caused it to sag downward into the mantle by an additional 8,000 m. In total, Mauna Loa is a 17,170 m thick accumulation of rock.
_](figures/11-volcanism/figure-11-22.png)
Figure 11.22: Comparison of volcano sizes and shapes. Broad, rounded shield volcanoes are the largest, followed by cone-shaped composite volcanoes. Straight-sided cinder cones are the smallest, and barely visible in the scale of the diagram. Source: Karla Panchuk (2017) CC BY 4.0 modified after Steven Earle (2015) CC BY 4.0 view original
Kīlauea Volcano is also a shield volcano, albeit a much flatter one. Kīlauea Volcano rises only 18 m about the surrounding terrain, and is almost not visible in the scale of the diagram, however it still stretches over a distance of 125 km along the eastern side of the Island of Hawai‘i.
Composite volcanoes are the next largest. Mt. St. Helens is shown on the left of Figure 11.22. It rises 1,356 m above the surrounding terrain in the Cascade Range of the western United States, and has a diameter of approximately 6 km. Composite volcanoes tend to be no more than 10 km in diameter. Unlike shield volcanoes, composite volcanoes have a distinctly conical shape, with sides that steepen toward the summit.
Cinder cones are the smallest, and almost too small to see next to a volcano like Mauna Loa. Eve Cone is a cinder cone on the flanks of Mt. Edziza in northwestern British Columbia. It rises 172 m above the landscape, and has a diameter of under 500 m. Cinder cones have straight sides, unlike upward-steepening composite volcanoes, or rounded shield volcanoes.
11.3.1 Volcano Structure
11.3.1.1 Shield Volcanoes
Shield volcanoes, like the Sierra Negra volcano in the Galápagos Islands (Figure 11.23, top), get their gentle hill-like shape because they are built of successive flows of low-viscosity basaltic lava (Figure 11.23, bottom). The low viscosity of the lava means that it can flow for long distances, resulting in the greater size of shield volcanoes compared to composite volcanoes or cinder cones.
. Bottom- Karla Panchuk (2017) CC BY 4.0 _](figures/11-volcanism/figure-11-23.png)
Figure 11.23: Shield volcano. Top: The Sierra Negra volcano in the Galápagos Islands exhibits the low, rounded shape characteristic of shield volcanoes. Bottom: Diagram of a shield volcano island, showing the build up of basaltic lava flows. Sources: Top- BRJ INC. (2012) CC BY-NC-ND 2.0 view source. Bottom- Karla Panchuk (2017) CC BY 4.0
11.3.1.2 Composite Volcanoes (Stratovolcanoes)
Composite volcanoes, like Cotopaxi in Figure 11.24 (top), consist of layers of lava alternating with layers of tephra (blocks, bombs, lapilli, and ash; Figure 11.24, bottom). The layers (strata) is where the alternative name, stratovolcano comes from. Cotopaxi displays the characteristic shape of composite volcanoes, which have slopes that get steeper near the top of the volcano. The change in the slope reflects the accumulation of tephra fragments near the volcano’s vent. Composite volcanoes typically erupt higher viscosity andesitic and rhyolitic lavas, which do not travel as far from the vent as basaltic lavas do. This results in volcanoes of smaller diameter than shield volcanoes. A notable exception is Mt. Fuji in Japan, which erupts basaltic lava.
. Click the image for more attributions. _](figures/11-volcanism/figure-11-24.png)
Figure 11.24: Composite volcano. Top: Cotopaxi in Ecuador exhibits the upward-steepening cone characteristic of composite volcanoes. Bottom: Diagram of a composite volcano showing alternating layers of lava and tephra. Sources: Karla Panchuk (2017) CC BY 4.0; Top photo by Simon Matzinger (2014) CC BY 2.0 view source. Click the image for more attributions.
From a geological perspective, composite volcanoes tend to form relatively quickly and do not last very long. If volcanic activity ceases, it might erode away within a few tens of thousands of years. This is largely because of the presence of pyroclastic eruptive material, which is not strong.
11.3.1.3 Cinder Cones (Spatter Cones)
Cinder cones, like Mt. Capulin in Figure 11.25, have straight sides and are typically less than 200 m high. Most are made up of fragments of scoria (vesicular rock from basaltic lava) that were expelled from the volcano as gas-rich magma erupted. Because cinder cones are made up almost exclusively of loose fragments, they have very little strength. They can be eroded away easily, and relatively quickly.
. Click the image for more attributions._](figures/11-volcanism/figure-11-25.png)
Figure 11.25: Cinder cone. These small, straight-sided volcanoes are made of volcanic fragments ejected when gas-rich basaltic lava erupts. Sources: Karla Panchuk (2017) CC BY 4.0, with photograph by R. D. Miller, U. S. Geological Survey (1980) Public Domain view source. Click the image for more attributions.
11.4 Types of Volcanic Eruptions
Volcanoes produce a variety of materials when they erupt. The characteristics of the eruptions themselves also vary from one volcano to the next, and sometimes from one eruption to the next for the same volcano. Eruptions are classified according how explosive they are, and the height of their eruption column- how high they blast material into the air.
Both the explosiveness of an eruption and the height of the eruption column are related in part to the composition of magma and the amount of gas it contains. In particular, magmas with more silica erupt more explosively. The higher the silica content, the greater the viscosity of the magma. This means more pressure can build up before the magma is forced out of the volcano. Magma with more silica also tends to contain more dissolved gas. The gas helps to propel magma out of the volcano, in the same way that the bubbles in a shaken bottle of pop cause the pop to foam out when the lid is removed.
There are four types of eruptions with properties determined mostly by the silica content of magma, and the amount of gas it contains. In order of increasing explosiveness, these are Hawai’ian, Strombolian, Vulcanian, and Plinian eruptions. Any composition of magma can have an explosive eruption if the magma suddenly encounters water. Hot magma contacting groundwater or seawater causes the water to flash to steam. Explosive eruptions driven by water are called hydrovolcanic (or phreatic) eruptions.
11.4.1 Hawai‘ian Eruptions
Hawai‘ian eruptions are named after the characteristic eruptions of volcanoes of the Hawai‘ian islands. Hawai‘ian eruptions are effusive (flowing) rather than explosive because they erupt low-viscosity basaltic lava. Hawai‘ian eruptions form shield volcanoes and can also take the form of fissure eruptions. Fissure eruptions occur when lava erupts from long cracks in the ground rather than from a central vent.
Figure 11.26 shows examples from two eruptions on of Hawai‘i. In the upper left and right are images from the November 1959 eruption of Kīlauea Iki Crater. The upper left shows a fissure eruption and effusive flow of lava. Burning trees appear as bright spots toward the bottom of the photo. Figure 11.26 (right) shows a lava fountain reaching 425 m above Kīlauea Iki Crater. U. S. Geological Survey scientists reported that volcanic bombs up to 60 cm across smashed the guard rail and dented the asphalt on the road. Figure 11.26 (lower left) shows Hawaiian Volcano Observatory (HVO) scientists making a quick getaway, with lava fountains from Mauna Loa Volcano in the background.
 Bottom left: R. B. Moore, U. S. Geological Survey (1984) Public Domain. [view source](https://flic.kr/p/qP8tH2) Right- U. S. Geological Survey (1959) Public Domain. [view source](https://volcanoes.usgs.gov/volcanoes/kilauea/geo_hist_kilauea_iki.html) _](figures/11-volcanism/figure-11-26.png)
Figure 11.26: Hawai‘ian eruptions. Top left: Fissure eruption at Kīlauea Iki Crater in November of 1959. Bottom left: Lava fountains from an eruption of Mauna Loa Volcano in 1984. Right: Lava fountain from Kīlauea Iki Crater eruption in November of 1959. Sources: Top left- U. S. Geological Survey (1959) Public Domain. view source Bottom left: R. B. Moore, U. S. Geological Survey (1984) Public Domain. view source Right- U. S. Geological Survey (1959) Public Domain. view source
The photographs of the Kīlauea Iki Crater and Mauna Loa Volcano eruptions make the point that while Hawai‘ian eruptions are considered “gentle” eruptions, this is a relative term. “Gentle” eruptions range from lava flows that can be safely sampled by trained personnel, as in Figure 11.5, to lava fountains that soar hundreds of metres above the tree tops and rain large and dangerous rocks upon the surroundings.
11.4.2 Strombolian Eruptions
Strombolian eruptions, named for Mt. Stromboli in Italy, occur when basaltic lava has higher viscosity and higher gas content. The sticky lava is ejected in loud, violent, but short-lived spattery eruptions. Clumps of gas-rich lava thrown 10s to 100s of metres in the air accumulate as scoria in a pile around the vent, forming cinder cones. Figure 11.27 shows a strombolian eruption in the crater of Mt. Etna. A smaller cinder cone is forming around the vent as lava sputters out of it.
 Click the image for more attributions. _](figures/11-volcanism/figure-11-27.png)
Figure 11.27: Strombolian eruption of Mt. Etna. Sputtering lava forms a smaller cinder cone around a vent within the crater of Etna. Source: Karla Panchuk (2017) CC BY-SA 4.0. Photograph- Robin Wylie (2012) CC BY 2.0. view source Click the image for more attributions.
11.4.3 Vulcanian Eruptions
Vulcanian eruptions get their name from the volcanic Italian island of Vulcano, which itself takes the name of the Roman god of fire, Vulcan. In Roman mythology, Vulcan was the maker of armour and weaponry for the gods, and volcanic eruptions were attributed to him working in his forge.
Vulcanian eruptions are far more explosive than Strombolian eruptions, and can blast tephra and gas to a height of 5 to 10 km. The explosiveness is related to a build-up of pressure as the higher viscosity of intermediate silica content lava restricts the escape of gas. Vulcanian eruptions produce large quantities of ash in addition to blocks and bombs.
The Vulcanian eruption of Mt. Pelée on the island of Martinique in 1902 resulted in the first detailed documentation by geologists of a devastating phenomenon that is now referred to as a pyroclastic flow (Figure 11.28). Volcanic debris from the collapse of a lava dome on Mt. Pelée combined with hot gas to form a searing avalanche that raced down the mountain, over the city of St. Pierre, and into the harbour.
 Click the image for more attributions._](figures/11-volcanism/figure-11-28.png)
Figure 11.28: A series of photos taken by Alfred Lacroix during the eruption of Mt. Pelée on May 8, 1902 showing the development of the pyroclastic flow that destroyed the city of St. Pierre and nearly 30,000 inhabitants. Source: Karla Panchuk (2017) CC BY 4.0. Photograph: Alfred Lacroix (1902) Public Domain. view original Click the image for more attributions.
The French geologist Alfred Lacroix described what he saw as a “nuée ardente,” or thick fiery cloud. The following first-hand account was published in Cosmopolitan Magazine in July of 1902, attributed to Ellery S. Scott, a sailor on the steamship Roraima:
"In idle interest, I turned my glass toward Mont Pelee. It was at that very moment that the whole top of the mountain seemed blown into the air. The sound that followed was deafening. A great mass of flames, seemingly a mile in diameter, with twisting giant wreaths of smoke, rolled thousands of feet into the air, and then overbalanced and came rolling down the seamed and cracked sides of the mountain. Foot hills were overflowed by the onrushing mass. It was not mere flame and smoke. It was molten lava, giant blocks of stone and a hail of smaller stones, with a mass of scalding mud intermingled.
For one brief moment I saw the city of St. Pierre before me. Then it was blotted out by the overwhelming flood. There was no time for the people to flee. They had not even time to pray…. I had called to Carpenter Benson to start the windlass, but before he could move, the “Roraima” rolled almost on her port beam-ends, and then as suddenly went to starboard. The funnel, masts and boats went by the board in an instant. The decks were swept clean. The hatches were staved in. The next instant a hail of fire and red-hot stones was upon the ship. Then came the scalding mud. The saloon was ablaze. The ship seemed doomed. Men were struck down all around me by flaming masses of lava. From bright sunlight the air became dense as midnight. The smoke that rolled down from the crater’s mouth had blotted the sun from our vision."
Scott’s account vividly describes of the speed of the pyroclastic flow. In some cases, pyroclastic flows travel at speeds greater than 700 km/h. They are able to travel rapidly because they behave like a fluid, and can also ride on a cushion of hot gas. Scott says the city was “blotted out by a flood,” yet the lower parts of buildings remained (Figure 11.29), and human remains were found in streets and homes where they had fallen. The ruins of St. Pierre look as though the top of the city were shaved off, and that is effectively what happened as the pyroclastic flow rushed across it, buoyed by gas.
; Bottom- Boston Public Library (2013) CC BY 2.0 [view source](https://flic.kr/p/ke3gz6)_](figures/11-volcanism/figure-11-29.png)
Figure 11.29: Two stereographs of the ruins of St. Pierre, published in 1902. Stereographs are viewed with a stereoscope to make an image appear three dimensional. Top- “St. Pierre, ‘the city of dead,’ Mt. Pelee smoking, Martinique”; Bottom- “Overlooking the mud-filled Roxelane River bed, and ash-covered ruins, to Mont Pelée, St. Pierre, Martinique.” Source: Top- Boston Public Library (2013) CC BY 2.0 view source; Bottom- Boston Public Library (2013) CC BY 2.0 view source
The vast majority of fatalities from the eruption were caused by the heat of pyroclastic flow. Examination of the ruins of St. Pierre revealed that glass had melted, but copper had not, putting the temperature at between 700 ºC and 1000 ºC (1292 ºF to 1832 ºF).
11.4.4 Plinian Eruptions
Plinian eruptions are explosive eruptions of intermediate to felsic lava, and can form eruptive columns up to 45 km high. The origin of the name is the eruption of Vesuvius in 79 CE, which buried the towns of Pompeii and Herculaneum. The Roman admiral Gaius Plinius Secundus, also known as Pliny the Elder, attempted a rescue mission when he saw the column of ash and debris above Vesuvius, but died of unknown causes without being able to reach Herculaneum.
A more recent Plinian eruption was that of Mt. Redoubt on April 21, 1990, shown in Figure 11.30. Pyroclastic flows resulted, as did lahars, landslides that formed when glaciers melted and turned volcanic ash into mud. The shape of the eruptive column, with parts of the column appearing to spread out in flat layers at different levels, reflects differences in atmospheric characteristics.
 Click the image for more attributions._](figures/11-volcanism/figure-11-30.png)
Figure 11.30: Plinian eruption of Mt. Redoubt in Alaska on April 21, 1990. Source: Karla Panchuk (2017) CC BY 4.0. Photograph: R. Clucas, U. S. Geological Survey (1990) Public Domain. view source Click the image for more attributions.
11.4.5 Hydrovolcanic (Phreatic) Eruptions
Hydrovolcanic eruptions can be far more explosive than Plinian eruptions. They occur when water in the form of groundwater, seawater, or even melting glacial ice or snow comes into contact with magma. Heat from the magma changes water suddenly to steam, which can expand to more than a thousand times the original volume of water. The sudden expansion results in an explosive force that can blast a volcano to pieces and create large amounts of volcanic ash.
In April of 2010, activity by the Icelandic volcano Eyjafjallajökull (Figure 11.31) melted the glacier above it, releasing large quantities of water and triggering a hydrovolcanic eruption. Ash rose in a plume 10 km high, and was blown westward and into the skies over Europe. Volcanic ash can damage or destroy aircraft engines, so the precaution was taken to prohibit air travel for a 5-day period. The enormous economic impact of stopping flights has led to numerous studies about the best way to deal with similar events with volcanic ash in the future.
Right photograph: Henrik Thorburn (2010) CC BY 3.0 [view source](https://commons.wikimedia.org/wiki/File:Eyjafjallajokull_volcano_plume_2010_04_17.jpg) Click the image for more attributions._](figures/11-volcanism/figure-11-31.png)
Figure 11.31: Hydrovolcanic eruption of Eyjafjallajökull in April of 2010. Left- Eruptive column with volcanic lightning. Volcanic lightning is caused by the static electricity generated by volcanic ash particles rubbing together. Right- Another view of the ash cloud, with westward winds carrying ash toward Europe where it would disrupt air traffic. Source: Karla Panchuk (2017) CC BY-SA 4.0. Left photograph: Terje Sørgjerd (2010) CC BY-SA 3.0 view sourceRight photograph: Henrik Thorburn (2010) CC BY 3.0 view source Click the image for more attributions.
11.5 Plate Tectonics and Volcanism
Thus far volcanoes have been discussed in terms of the kinds of volcanic mountains they form, the materials they produce, and the style of eruption they have. All of these characteristics can be tied together into a big picture by considering the plate tectonic settings in which magma forms (Figure 11.32). The vast majority of volcanoes are present along plate tectonic boundaries.
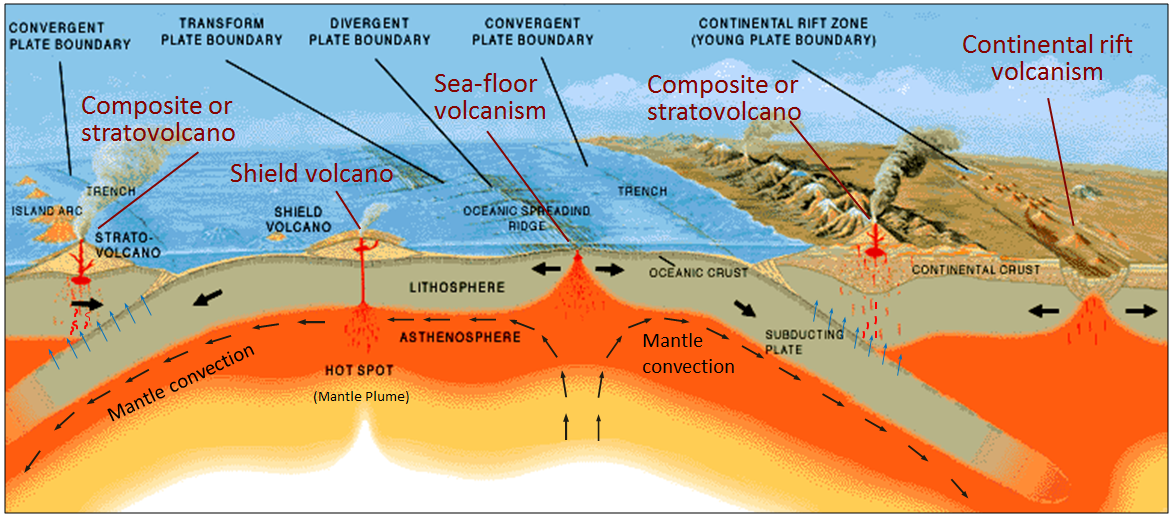 and U. S. Geological Survey (1999) Public Domain [view original](http://pubs.usgs.gov/gip/dynamic/Vigil.html)_](figures/11-volcanism/figure-11-32.png)
Figure 11.32: Plate tectonic settings of volcanism. Volcanoes along subduction zones are the result of flux melting (lowering the melting point by adding water). Decompression melting produces volcanoes along divergent margins (ocean spreading centres and continental rift zones), as well as above mantle plumes. Contact between hot mafic partial melts and felsic rocks can trigger partial melting of the felsic rocks (melting from conduction). Source: Karla Panchuk (2017) CC BY 4.0. Modified after Steven Earle (2015) CC BY 4.0 view original and U. S. Geological Survey (1999) Public Domain view original
There are four main scenarios to consider:
- Divergent boundaries where melting is triggered by decompression
- Subduction zones (ocean-ocean and ocean-continent convergent boundaries) where flux melting occurs as water is released from subducting ocean crust
- Hot spots where plumes of hot mantle material rise up, then melt as a result of decompression.
- Melting by conduction when magma transfers heat to rocks having a lower melting temperature.
11.5.1 Decompression Causes Volcanism Along Spreading Centres and Rift Zones
At an ocean spreading ridge (centre of Figure 11.32), convection moves hot mantle rock slowly upward at rates of cm per year. At roughly 60 km below the surface, the mantle rocks have decompressed is enough to permit partial melting of approximately 10% of the ultramafic rock. Mafic magma is produced, and it moves up toward the surface. Magma fills vertical fractures produced by the spreading and spills out onto the sea floor making pillow lavas and lava flows. Spreading-ridge volcanism is taking place approximately 200 km offshore from the west coast of Vancouver Island.
In continental rift zones where continental crust is thinning (far right in Figure 11.32), a similar decompression process occurs, triggering partial melting of ultramafic mantle rocks. However, if the continental crust above the region where melting occurs has a lower melting temperature than the mafic melt that is produced, the continental crust will also melt.
Continental rift zones can have a range of volcano types. If mafic magma erupts, shield volcanoes, broad lava flows, and cinder cones result. However, if rocks of other compositions are melted and added in, or the mafic magma undergoes fractional crystallization before erupting, then composite volcanoes will also form.
11.5.2 Water Causes Partial Melting Along Subduction Zones
At an ocean-continent convergent boundary (Figure 11.32, right) or ocean-ocean convergent boundary (Figure 11.32, left), oceanic crust is pushed down into the mantle. Although temperatures are high, the slab is kept from melting by high pressures. However, under these conditions minerals in the slab release water from within their crystal structures. The water lowers the melting point of rock above the slab, and partial melting is triggered within the mantle. Mafic magma rises through the mantle to the base of the crust. There it contributes to partial melting of crustal rock, and more felsic material is added to the magma. The magma, now intermediate in composition, continues to rise and assimilate crustal material. In the upper part of the crust, it accumulates into plutons. Over time, fractional crystallization of magma within the pluton can make it even more silica-rich. From time to time, the magma from the plutons rises toward surface, leading to volcanic eruptions.
Composite volcanoes with Vulcanian or Plinian eruption styles are characteristic of the volcanic arcs that form in subduction zones, although in the Trans-Mexico Volcanic Belt, Strombolian eruptions produce short-lived cinder cones. Where two margins of oceanic crust collide, the volcanic arc will be a chain of volcanic islands. Where continental and oceanic crust collide, there will be a volcanic arc on the continental crust.
11.5.2 Mt. St. Helens: A Composite Volcano in the Cascades Range Continental Volcanic Arc
On May 18, 1980 at 8:32 a.m., a M5.1 earthquake shook Mt. St. Helens, and marked the start of a 9-hour Plinian eruption (Figure 11.33) with a 24 km high eruption column and multiple pyroclastic flows. By the time the eruption was over, a large part of the volcano had been blasted away.
. Bottom left- R. Hoblitt, U. S. Geological Survey, Cascades Volcano Observatory (1979) Public Domain (label added) [view source](https://www.usgs.gov/media/images/aerial-photo-mount-st-helens-volcano-pre-1980-eruption). Bottom right- Steven Earle (2015) CC BY 4.0 (label added) [view source](https://opentextbc.ca/physicalgeologyearle/wp-content/uploads/sites/145/2016/03/msh-3.jpg). Click the image for more attributions._](figures/11-volcanism/figure-11-33.png)
Figure 11.33: Eruption of composite subduction-zone volcano Mt. St. Helens on May 18, 1980. Top- Plinian eruption column. Bottom left- Mt. St. Helens before the eruption. Bottom right- The remains of Mt. St. Helens after the eruption. Sources: Top- Karla Panchuk (2017) CC BY 4.0; Top- Photograph by NOAA (1980) Public Domain view source. Bottom left- R. Hoblitt, U. S. Geological Survey, Cascades Volcano Observatory (1979) Public Domain (label added) view source. Bottom right- Steven Earle (2015) CC BY 4.0 (label added) view source. Click the image for more attributions.
The explosive eruption was driven by gas-rich rhyolitic magma, however not all of Mt. St. Helens’ eruptions have been of felsic or intermediate material. The lava tube in Figure 11.10 (bottom) is from a time when Mt. St. Helens erupted basaltic lava. Data from the iMUSH (Imaging Magma Under St. Helens) project show that a magma chamber is present beneath Mt. St. Helens at between 5 and 14 km depth, but that a much larger magma chamber is present below it, which extends down to the mantle (Figure 11.34). Earthquakes in the 24 hours after the 1980 eruption suggested movement of magma within the smaller chamber (yellow arrows in Figure 11.34), but earthquakes from 1980 to 2005 indicate movement of magma within the deeper chamber as well (black arrows).
 (Imaging Magma Under St. Helens) project results. In a 24 hour period after the May 18, 1980 eruption, earthquakes in and around the smaller magma chamber suggested migration of magma (yellow arrows). Earthquakes recorded between 1980 and 2005 suggest migration of magma within a larger chamber that extends to the mantle (black arrows). The larger magma chamber might feed another smaller chamber beneath the Indian Heaven Volcanic Field. _Source: Karla Panchuk (2017) CC BY 4.0, based on Kiser et al. (2016), Figure 4b](figures/11-volcanism/figure-11-34.png)
Figure 11.34: Magma chambers beneath Mt. St. Helens and Indian Heaven Volcanic Field, sketched from iMUSH (Imaging Magma Under St. Helens) project results. In a 24 hour period after the May 18, 1980 eruption, earthquakes in and around the smaller magma chamber suggested migration of magma (yellow arrows). Earthquakes recorded between 1980 and 2005 suggest migration of magma within a larger chamber that extends to the mantle (black arrows). The larger magma chamber might feed another smaller chamber beneath the Indian Heaven Volcanic Field. _Source: Karla Panchuk (2017) CC BY 4.0, based on Kiser et al. (2016), Figure 4b
The complex history of Mt. St. Helens could reflect changes in the composition of magma within the small chamber over time, as fractionation proceeds, and the magma becomes more silica rich. However, movement of more mafic magma from the larger chamber could also contribute to eruptions with different chemical compositions. The larger magma chamber may be connected to a chamber feeding the nearby Indian Heaven Volcanic Field, which contains shield volcanoes and cinder cones, and for which basalt makes up 80% of erupted materials.
11.5.3 Mantle Plumes Can Cause Volcanism Away from Plate Boundaries
__Mantle plumes __are rising columns of hot solid rock. The column may be kilometres to 10s of kilometres across, but near the surface it spreads out to create a mushroom-like head that is 10s to over 100 kilometres across. Mantle plumes are different from the convection that normally occurs beneath ocean spreading centres: plumes rise approximately 10 times faster than mantle convection normally occurs, and may originate deep in the mantle, possibly just above the core-mantle boundary.
When the mantle plume rises to the base of the lithosphere, the pressure is low enough to permit partial melting of the plume material, producing mafic magma. Heat carried by the mantle plume may also melt rock adjacent to the plume. The magma rises and feeds hotspot volcanoes. The lithospheric plate above the mantle plume is moving across the plume, so a chain of hotspot volcanoes can result as existing hotspot volcanoes are slowly moved away from the mantle plume, and new volcanoes form in the lithosphere.
Many shield volcanoes are associated with mantle plumes, including those that make up the Hawai’ian islands. All of the Hawai’ian volcanoes are related to the mantle plume that currently lies beneath Mauna Loa, Kilauea, and Lōʻihi(Figure 11.35, top). There is evidence of crustal magma chambers beneath all three active Hawai’ian volcanoes. At Kīlauea, the magma chamber appears to be several kilometres in diameter, and is situated between 8 km and 11 km below surface (Lin et al., 2014). In this area, the Pacific Plate is moving northwest at a rate of about 7 cm/year. This means that the earlier formed — and now extinct — volcanoes have now moved well away from the mantle plume. The hotspot has in fact been present for at least 85 million years (Regelous et al., 2003), as evidenced by the long chain of eroded and submerged mountains stretching to the Aleutian Trench (Figure 11.35, bottom).
. Bottom- National Geophysical Data Center/ U. S. Geological Survey (2006) Public Domain (labels added) [view source](https://commons.wikimedia.org/wiki/File:Hawaii_hotspot.jpg)._](figures/11-volcanism/figure-11-35.png)
Figure 11.35: Hawai’ian hotspot volcanoes and volcanic chain. Top- A mantle plume beneath Hawai’i supplies magma to Mauna Loa Volcano, Kīlauea Volcano, and Lōʻihi Seamount. Volcanoes to the northwest are no longer active because they have moved away from the plume. Bottom- Bathymetric (depth) map showing the chain of islands stretching toward the Aleutian Trench, and marking the progress of the Pacific Plate over the mantle plume. Source: Top- J. E. Robinson, U. S. Geological Survey (2006) Public Domain view source. Bottom- National Geophysical Data Center/ U. S. Geological Survey (2006) Public Domain (labels added) view source.
Kīlauea Volcano is approximately 300 ka old, while neighbouring Mauna Loa Volcano is over 700 ka and Mauna Kea Volcano is over 1 Ma. If volcanism continues above the Hawaii mantle plume in the same manner that it has for the past 85 Ma, it is likely that Kīlauea Volcano will continue to erupt for at least another 500,000 years. By that time, its neighbour, Lōʻihi Seamount, will have emerged from the sea floor, and its other neighbours, Mauna Loa and Mauna Kea, will have become significantly eroded, like their cousins, the islands to the northwest.
11.5.3.1 Large Igneous Provinces (LIPs)
While the Hawaii mantle plume has produced a relatively low volume of magma for approximately 85 Ma, other mantle plumes are less consistent, and some generate massive volumes of magma over relatively short time periods. Although their origin is still controversial, it is thought that the volcanism leading to large igneous provinces (LIPs) is related to very high volume but relatively short duration bursts of magma from mantle plumes. An example of an LIP is the Columbia River Basalt Group, which extends across Washington, Oregon, and Idaho in the United States (Figure 11.36). This volcanism, which covered an area of about 160,000 km2 with basaltic rock up to several hundred metres thick, took place between 17 and 14 Ma.
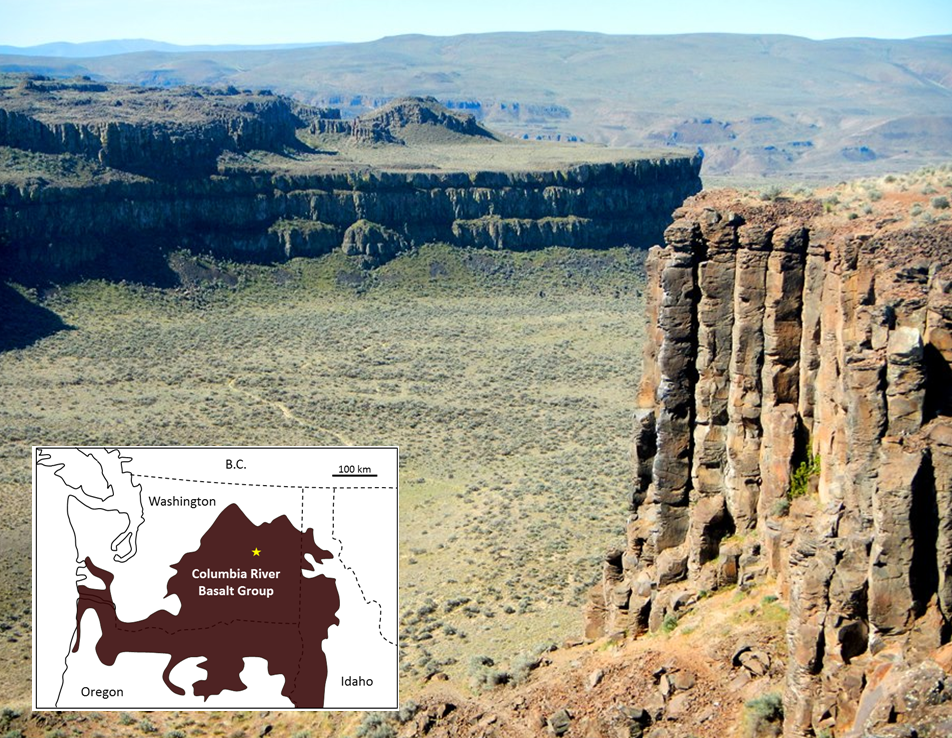_](figures/11-volcanism/figure-11-36.png)
Figure 11.36: Part of the Columbia River Basalt Group at Frenchman Coulee, eastern Washington, United States. All of the flows visible here have formed large (up to two metres in diameter) columnar basalts, a result of relatively slow cooling of flows that are tens of m thick. The inset map shows the approximate extent of the 17 to 14 Ma Columbia River Basalts, with the location of the photo shown as a star. Source: Steven Earle (2015) CC BY 4.0 view source
The mantle plume that is assumed to be responsible for the Columbia River LIP is now situated beneath the Yellowstone area, where it leads to felsic volcanism. Over the past 2 Ma, three very large explosive eruptions at Yellowstone have yielded approximately 900 km3 of felsic magma. This is approximately 900 times the volume of the 1980 eruption of Mt. St. Helens, but only 5% of the volume of mafic magma in the Columbia River LIP.
Most other LIP eruptions are much bigger. The Siberian Traps (also basalt), which erupted at the end of the Permian period at 251 Ma, are estimated to have produced approximately 40 times as much lava as the Columbia River LIP. The largest known LIP is the Ontong Java Plateau, located in the southwest Pacific Ocean. It formed at around 122 Ma, and presently covers 1,500,000 km2 and has a volume of 5,000,000 km3. But this is only a small fraction of its original size. The majority of it has been subducted, and it may have been split into pieces that have been classified as separate LIPs.
11.5.3.2 Kimberlites
Kimberlite pipes are carrot-shaped cones of ultramafic rock. They form from the explosive eruption of mantle plumes originating at depths of 150 to 450 km in the mantle. The plume makes its way to the surface quickly (over hours to days), having little interaction with the surrounding rocks, and thus preserving a sample of the ultramafic mantle. As the plume nears the surface, a build-up of gas causes it to pick up speed, and by the time it reaches the surface it may be travelling faster than the speed of sound. The explosiveness of kimberlite eruptions means that they do not form volcanic mountains on the surface, but leave circular holes in the ground.
Kimberlite eruptions that originate at depths greater than 200 km beneath old, thick, continental crust travel through the region of the mantle where diamond is stable. In some cases, such as in Saskatchewan and the Northwest Territories, kimberlites bring diamond-bearing material to the surface. All of Earth’s diamond deposits are thought to have originated in this way.
Diamond mines in kimberlites, such as the Ekati Mine in the Northwest Territories, are easy to spot by the characteristic circular hole that develops as miners excavate the cone-shaped structure (Figure 11.37). The kimberlites at Ekati erupted between 45 and 60 Ma. Many kimberlites are older, and some much older. There have been no kimberlite eruptions in historic times. The youngest known kimberlites are in the Igwisi Hills in Tanzania and are only about 10,000 years old. The next youngest date to approximately 30 Ma.
. Click the image for more attributions._](figures/11-volcanism/figure-11-37.png)
Figure 11.37: The Ekati diamond mine in the Northwest Territories, part of the Lac de Gras kimberlite field. Source: Karla Panchuk (2017) CC BY-SA 4.0; Photograph by J. Pineau (2010) CC BY-SA 3.0 view source. Click the image for more attributions.
11.6 Volcanic Hazards
The basaltic lava flows produced by volcanoes on the island of Hawai’i are responsible for extensive damage to homes, infrastructure, and habitats. Figure 11.38 shows lava flows (in black) from the Puʻu ʻŌʻō crater of Kīlauea Volcano. The lava flow destroyed the house, and is encroaching on the transfer station. Smoke in the background marks locations where additional flows have broken out and are burning vegetation.
_](figures/11-volcanism/figure-11-38.jpg)
Figure 11.38: Lava flow from Kīlauea’s Puʻu ʻŌʻō crater. Lava (in black) has destroyed a house and threatens a transfer station. Source: U. S. Geological Survey (2014) Public Domain view source
In spite of the damage that lava flows can cause, they are not the volcanic hazard with the greatest impact on lives and infrastructure. Even the relatively free-flowing Hawai’ian basaltic lava moves slowly enough that it can be escaped on foot. Far more dangerous hazards are related to gases and volcanic debris. However, the largest impact and the greatest suffering are caused not by the immediate effects of volcanic eruptions, but by large-scale changes to climate and environments caused by volcanism. Indirect effects resulting in respiratory distress, toxicity, famine, and habitat destruction have accounted for approximately 8 million deaths during historical times, while direct effects have accounted for fewer than 200,000, or 2.5% of the total.
11.6.1 Volcanic Gas and Tephra Emissions
Large volumes of rock and gases are emitted during major Plinian eruptions at composite volcanoes, and a large volume of gas is released during some very high-volume effusive eruptions. Gases and fine particles of volcanic ash can cause respiratory distress and poisoning, and ash poses a risk for aircraft.
Most of the tephra from large explosive eruptions ascends high into the atmosphere, and some of it is distributed around Earth by high-altitude winds. The larger components (larger than 0.1 mm) fall closer to the volcano, and the accumulation of tephra from large eruptions can cause serious damage and casualties. When the large eruption of Mt. Pinatubo in the Philippines occurred in 1991, tens of centimetres of ash accumulated in fields and on rooftops in the surrounding populated region. Heavy typhoon rains hit the island at the same time and added to the weight of the tephra. The weight was too much for roofs to bear, and thousands of structures collapsed, causing at least 300 of the 700 deaths attributed to the eruption.
One of the long-term effects of adding volcanic particles and gases to the atmosphere is cooling. Over an eight-month period in 1783 and 1784, a massive effusive eruption took place at the Laki volcano in Iceland. Although there was relatively little volcanic ash involved, a massive amount of sulphur dioxide was released into the atmosphere, along with a significant volume of hydrofluoric acid (HF). The sulphur dioxide combined with water to make sulphate__ aerosols__, which block incoming solar energy. The accumulation of sulphate aerosols over that 8 months led to dramatic cooling in the northern hemisphere. There were serious crop failures in Europe and North America, and a total of 6 million people are estimated to have died from famine and respiratory complications. In Iceland, poisoning from the HF resulted in the death of 80% of sheep, and 50% of cattle. The ensuing famine, along with HF poisoning, resulted in more than 10,000 human deaths, about 25% of the population.
11.6.2 Pyroclastic Flows
In a typical explosive eruption at a composite volcano, the tephra and gases are ejected with explosive force and sent high up into the atmosphere. As the eruption proceeds, and the amount of gas in the rising magma starts to decrease, and less gas is supplied to the eruption column. Parts of the column will become denser than air, leading the column to collapse and flow downward along the flanks of the volcano (Figure 11.39), picking up speed as it cools.
_](figures/11-volcanism/figure-11-39.jpg)
Figure 11.39: The Plinian eruption of Mt. Mayon, Philippines in 1984. Although most of the eruption column is ascending into the atmosphere, pyroclastic flows are traveling down the sides of the volcano in several places. Warnings were issued in time to evacuate 73,000 people. Source: C. G. Newhall, U. S. Geological Survey (1984) Public Domain view source
Pyroclastic flows can travel over water, in some cases for many kilometres. In 1902 the pyroclastic flow from the eruption of Mt. Pelée traveled out into the harbour and destroyed several wooden ships anchored there. The pyroclastic flow from the 1883 eruption of Krakatau traveled 80 km across the Sunda Straits and claimed victims on the southwest coast of Sumatra. It also triggered a tsunami.
One of the most famous pyroclastic flows occurred when Mt. Vesuvius erupted in 79 CE. It buried the cities of Pompeii and Herculaneum, killing an estimated 18,000 people.
11.6.3 Lahar
A lahar is any mudflow or debris flow that is related to a volcano (Figure 11.40). Most are caused by melting snow and ice during an eruption, as was the case with the lahar that destroyed the Colombian town of Armero in 1985 when the volcano Nevado del Ruiz caused the ice dam on a glacial lake to fail. The resulting lahar killed 23,000 in Armero, about 50 km from the volcano.
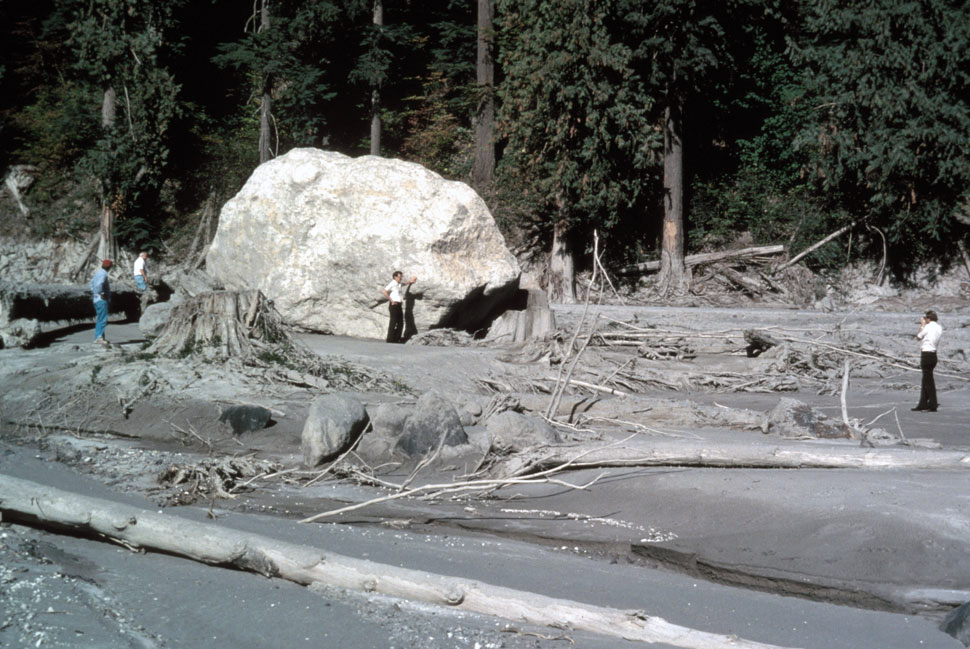_](figures/11-volcanism/figure-11-40.jpg)
Figure 11.40: Mud left behind from the lahar after the May 18, 1980 eruption of Mt. St. Helens. The lahar carried the boulder to its present location. Source: L. Topinka, U. S. Geological Survey (1980) Public Domain view source
Lahars can also happen when there is no volcanic eruption, because composite volcanoes tend to be weak and easily eroded. In October 1998, category 5 hurricane Mitch slammed into the coast of Central America. Damage was extensive and 19,000 people died. Fatalities were largely because of mudflows and debris flows triggered by intense rainfall — some regions received almost 2 m of rain over a few days.
At Casita Volcano in Nicaragua, the heavy rains weakened rock and volcanic debris on the upper slopes, resulting in a debris flow that rapidly built in volume as it raced down the steep slope. It struck the towns of El Porvenir and Rolando Rodriguez killing more than 2,000 people. El Porvenir and Rolando Rodriguez were new towns that had been built without planning approval in an area that was known to be at risk of lahars.
11.6.4 Sector Collapse and Debris Avalanche
In the context of volcanoes, sector collapse or flank collapse is the catastrophic failure of a significant part of an existing volcano, creating a large debris avalanche. This hazard was first recognized with the failure of the north side of Mt. St. Helens immediately prior to the large eruption on May 18, 1980.
In the weeks before the eruption, a large bulge had formed on the side of the volcano (Figure 11.41) as magma moved from depth into a magma chamber within the mountain itself. Early on the morning of May 18, a moderate earthquake struck and destabilized the bulge, leading to Earth’s largest observed landslide in historical times. The failure of this part of the volcano exposed the underlying magma chamber, causing it to explode sideways. This in turn exposed the conduit leading to the magma chamber below, resulting in a Plinian eruption lasting nine hours.
_](figures/11-volcanism/figure-11-41.jpg)
Figure 11.41: Bulge forming on the north side of Mt. St. Helens, April 27 1980. Source: P. Lipman, U. S. Geological Survey (1980) Public Domain view source
11.7 Monitoring Volcanoes and Predicting Eruptions
In 2005 U. S. Geological Survey geologist Chris Newhall made a list of the six most important signs of an imminent volcanic eruption. They are:
- Gas leaks — the release of gases (mostly H2O, CO2, and SO2) from the magma into the atmosphere through cracks in the overlying rock
- Bulging — the deformation of part of the volcano, indicating that a magma chamber at depth is swelling or becoming more pressurized
- Seismicity — many (hundreds to thousands) of small earthquakes, indicating that magma is on the move. The quakes may be the result of the magma forcing the surrounding rocks to crack, or a harmonic vibration that is evidence of magmatic fluids moving underground.
- Seismicity ceases — a sudden decrease in the rate of earthquake activity. This may indicate that magma has stalled, and that something is about to give way
- Big bump — a pronounced bulge on the side of the volcano (like the one at Mt. St. Helens in 1980), which may indicate that magma has moved close to surface
- Steam — steam eruptions ( phreatic eruptions) that happen when magma near the surface heats groundwater to the boiling point. The water eventually explodes, sending fragments of the overlying rock far into the air. With these signs in mind, it is possible to determine the necessary equipment to have and actions to take to monitor a volcano and predict when it might erupt. we can make a list of the equipment we should have and the actions we can take to monitor a volcano and predict when it might erupt.
11.7.1 Assessing Seismicity
The simplest and cheapest way to monitor a volcano is with seismometers, instruments that detect vibration. In an area with several volcanoes that have the potential to erupt (e.g., the Squamish-Pemberton area), a few well-placed seismometers can provide an early warning that something is changing beneath one of the volcanoes. There are currently enough seismometers in the Lower Mainland and on Vancouver Island to provide this information. You can view a map of Canadian National Seismograph Network here.
If there is seismic evidence that a volcano is coming to life, more seismometers should be placed in locations within a few tens of kilometres of the source of the activity (Figure 11.42). This will allow geologists to determine the exact location and depth of the seismic activity so that they can see where the magma is moving.
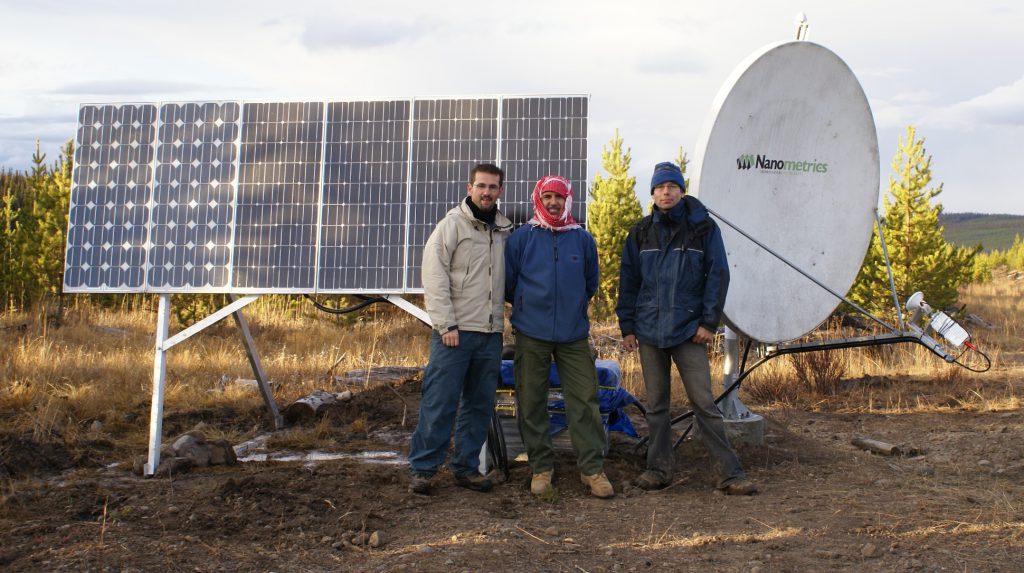
Figure 11.42: A seismometer installed in 2007 in the vicinity of the Nazco Cone, BC. Source: Cathie Hickson (n.d.) used with permission.
11.7.2 Detecting Gases
Water vapour quickly turns into clouds of liquid water droplets and is relatively easy to detect just by looking, but CO2 and SO2 are not as obvious. It’s important to be able to monitor changes in the composition of volcanic gases, and we need instruments to do that. Some can be monitored from a distance (from the ground or even from the air) using infrared devices, but to obtain more accurate data, we need to sample the air and do chemical analysis. This can be achieved with instruments placed on the ground close to the source of the gases, or by collecting samples (Figure 11.43) and analyzing them in a lab.
_](figures/11-volcanism/figure-11-43.jpeg)
Figure 11.43: A geologist collects a gas sample from Sherman Crater, Mt. Baker, Washington. Gas is drawn through a titanium tube inserted in a fumarole, and collected in a glass vacuum flask. Source: D. Tucker, U. S. Geological Survey (2006) Public Domain view source
11.7.3 Measuring Deformation
There are two main ways to measure ground deformation at a volcano. One is known as a tiltmeter, which is a sensitive three-directional level that can sense small changes in the tilt of the ground at a specific location. Another is through the use of GPS (global positioning system) technology (Figure 11.27). GPS is more effective than a tiltmeter because it provides information on how far the ground has actually moved — east-west, north-south, and up-down.
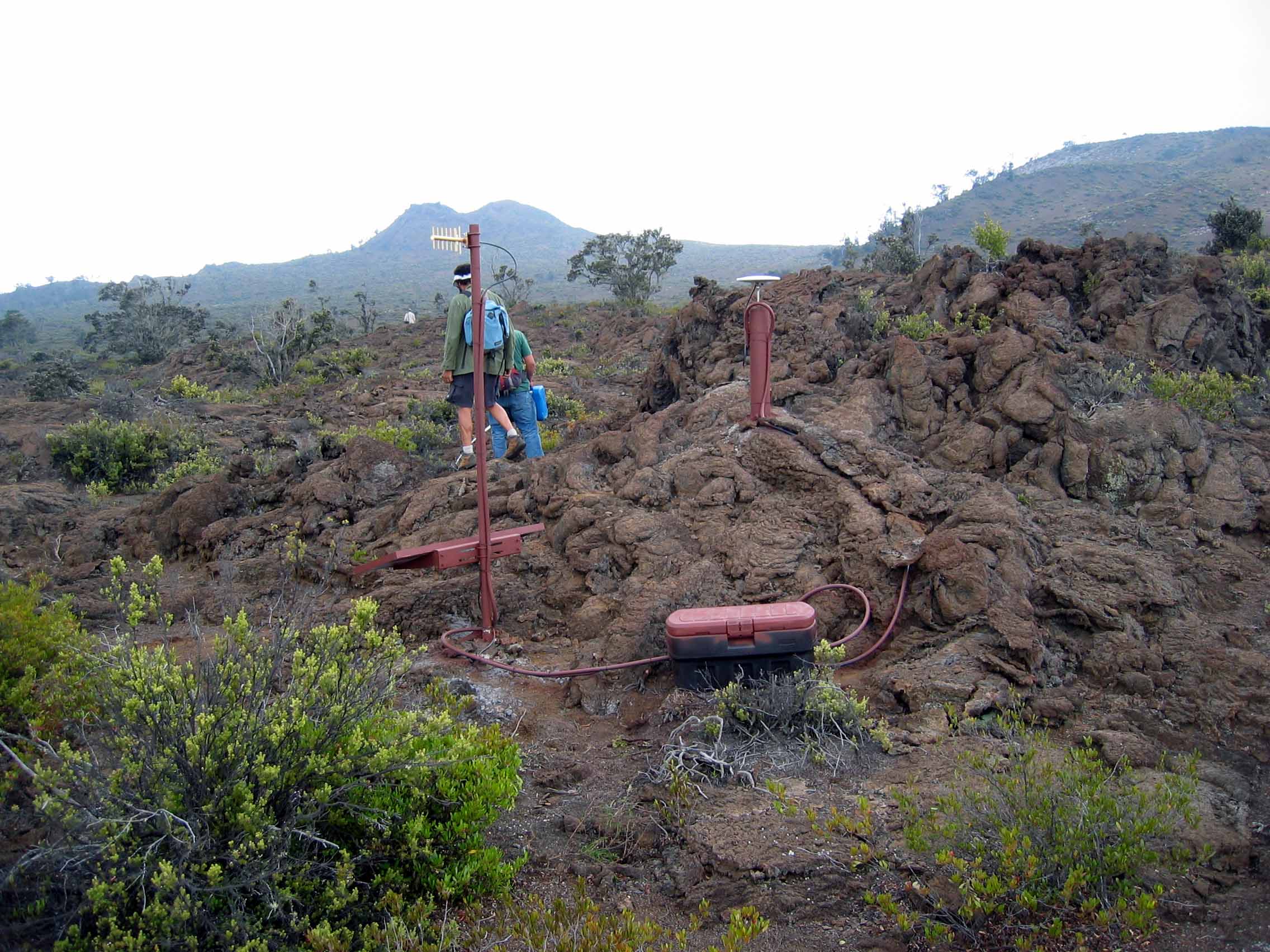_](figures/11-volcanism/figure-11-44.jpg)
Figure 11.44: A GPS unit installed at Hualālai Volcano, Hawaii. The dish-shaped antenna on the right is the GPS receiver. The antenna on the left is for communication with a base station. Source: U. S. Geological Survey (n.d.) Public Domain view source
11.7.4 Putting It All Together
By combining information from these types of sources, along with careful observations made on the ground and from the air, and a thorough knowledge of how volcanoes work, geologists can get a good idea of the potential for a volcano to erupt in the near future (months to weeks, but not days). They can then make recommendations to authorities about the need for evacuations and restricting transportation corridors.
Our ability to predict volcanic eruptions has increased dramatically in recent decades because of advances in our understanding of how volcanoes behave and in monitoring technology. Providing that careful work is done, there is no longer a large risk of surprise eruptions, and providing that public warnings are issued and heeded, it is less and less likely that thousands will die from sector collapse, pyroclastic flows, ash falls, or lahars. Indirect hazards are still very real, however, and we can expect the next eruption like the one at Laki in 1783 to take an even greater toll than it did then, especially since there are now roughly eight times as many people on Earth.
11.8 Volcanoes in Canada
Canada’s volcanically active regions are located in British Columbia and the Yukon Territory (Figure 11.45). At least 49 eruptions have occurred within these regions in the last 10,000 years. There are five volcanic regions associated with three types of plate tectonic settings: a subduction zone, a mantle plume, and a continental rift zone.
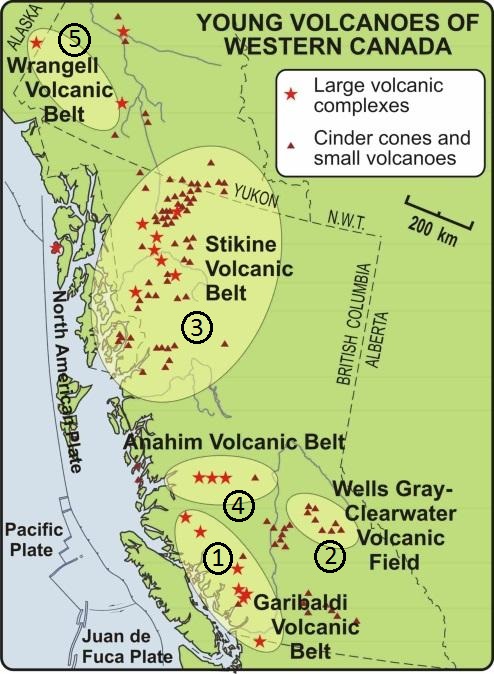 Click the image for copyright information._](figures/11-volcanism/figure-11-45.jpg)
Figure 11.45: Canada’s volcanic regions are located in British Columbia and the Yukon Territory. Volcanism is associated with three tectonic settings: the subduction zone along the west coast (Garibaldi Volcanic Belt, Wrangell Volcanic Belt), a continental rift zone (Wells Gray-Clearwater Volcanic Field, Stikine Volcanic Belt), and a mantle plume (Anahim Volcanic Belt). Source: Volcanoes Canada, Canadian Hazards Information Service, Natural Resources Canada (n.d.) view source Click the image for copyright information.
11.8.1 Subduction Zone Volcanism: Wrangell and Garibaldi Volcanic Belts
The Wrangell Volcanic Belt is the result of subduction beneath the North American Plate. Volcanoes in the Canadian part of the Wrangell Volcanic Belt erupted between 17.8 and 10.4 million years ago. They were fed by lava that seeped up along a leaky transform fault.
Southwestern British Columbia is at the northern end of the Juan de Fuca subduction zone, and part of the Cascade Volcanic Arc that extends south through Washington and Oregon. The Canadian part of the Cascade Arc has had a lower rate and volume of volcanism than U. S. portions. One reason is that the northern part of the Juan de Fuca Plate is subducting more slowly than the rest of the plate, or else has stalled.
The Garibaldi Volcanic Belt has several volcanic centres , or regions where volcanism has caused multiple volcanoes to develop (Figure 11.46).
. Click the image to enlarge._](figures/11-volcanism/figure-11-46.png)
Figure 11.46: Volcanic centres within the Garibaldi Volcanic Belt. The most recent eruption was 2,350 years ago at Mt. Meager. Source: Sémhur (2007) CC BY-SA 4.0 view source. Click the image to enlarge.
The most recent volcanic activity in this area was 2,350 years ago at Mt. Meager. An explosive eruption similar in magnitude to that of Mt. St. Helens in 1980 spread ash as far east as Alberta. There was also significant volcanic activity at Mt. Price and Mt. Garibaldi approximately 10,000 years ago as glacial ice receded. In both cases, lava and tephra built up against glacial ice. The western side of Mt. Garibaldi failed by sector collapse when the ice melted, leaving rocks unsupported. Eruption beneath glacial ice resulted in the formation of a tuya- a steep-sided, flat-topped volcano- called The Table near Mt. Garibaldi (Figure 11.47).
_](figures/11-volcanism/figure-11-47.jpg)
Figure 11.47: The Table, a tuya near Mt. Garibaldi. Tuyas form when volcanoes erupt beneath ice, and their shape is determined by rapid cooling beneath the ice sheet. Source: Andre Charland (2004) CC BY 2.0 view source
11.8.2 Mantle Plume Volcanism: Anahim Volcanic Belt
The chain of volcanic complexes and cones extending from Milbanke Sound to Nazko Cone is interpreted as being related to a mantle plume currently situated close to the Nazko Cone, just west of Quesnel (Figure 11.48). The North American Plate is moving in a westerly direction at about 2 cm per year with respect to this plume, and the series of now partly eroded shield volcanoes between Nazco and the coast is interpreted to have been formed by the plume as the continent moved over it.
 Click the image to enlarge._](figures/11-volcanism/figure-11-48.png)
Figure 11.48: Anahim Volcanic Belt, the result of a mantle plume beneath the North American Plate. Source: Sémhur (2007) CC BY-SA 4.0 view source Click the image to enlarge.
The Rainbow Range, which formed at approximately 8 Ma, is the largest of these older volcanoes. It has a diameter of about 30 km and an elevation of 2,495 m (Figure 11.49). The name “Rainbow” refers to the bright colours displayed by some of the volcanic rocks as they weather.
_](figures/11-volcanism/figure-11-49.jpg)
Figure 11.49: Tsitsutl, the “painted mountain” within the Rainbow Range of the Anahim Volcanic Belt. The vibrant colours of the Rainbow Range are the result of chemical weathering. Source: Drew Brayshaw (2015) CC BY-NC 2.0 view source
11.9 Summary
The topics covered in this chapter can be summarized as follows:
11.9.1 What Is A Volcano?
Volcanoes are places where molten rock escapes to Earth’s surface. Some volcanoes are cone-shaped or hill-shaped mountains, and some eruptions happen along fissures. Eruptions are fed by a magma chamber beneath the volcano. Sometimes a volcano collapses into empty space in the magma chamber beneath, forming a caldera.
11.9.2 Materials Produced by Volcanic Eruptions
Volcanoes produce gas, lava flows, and debris called tephra. The characteristics of a lava flows depend on whether the lava is thin and runny (mafic with low gas content) or thick and sticky (felsic with high gas content). Tephra is classified according to size. Ash is less than 2 mm in diameter, lapilli is between 2 mm and 64 mm, and blocks and bombs are larger than 64 mm.
11.9.3 Types of Volcanoes
Cinder cones are relatively small straight-sided volcanoes that are composed mostly of mafic rock fragments. Composite volcanoes consist of alternating layers of lava flows and tephra. The tend to be intermediate to felsic in composition, and get steeper toward the top. Shield volcanoes are broad, low, hill-like volcanoes that form from layers of low-viscosity mafic lava.
11.9.4 Types of Volcanic Eruptions
Volcanic eruptions can be classified according to how explosive they are, and how high into the atmosphere they blast material. Hawai’ian eruptions are relatively gentle effusive eruptions of low-viscosity mafic lava, and form shield volcanoes. Strombolian eruptions are more vigorous eruptions of mafic tephra. They blast material hundreds of metres into the air. The tephra falls out of the atmosphere to form a cinder cone. Vulcanian eruptions are explosive eruptions of intermediate composition lava, producing pyroclastic flows and eruptive columns from 5 to 10 km high. Plinian eruptions are highly explosive eruptions of felsic lava, and can produce eruption columns up to 45 km high. Both Vulcanian and Plinian eruptions are associated with composite volcanoes. Hydrovolcanic eruptions are the explosive result of magma or lava interacting with water, and rapidly changing the water to steam.
11.9.5 Plate Tectonics and Volcanism
Volcanism is closely related to plate tectonics. Most volcanoes are associated with convergent plate boundaries (at subduction zones), but a great deal of volcanic activity also occurs at divergent boundaries and areas of continental rifting. At convergent boundaries magma is formed where water from a subducting plate acts as a flux to lower the melting temperature of the adjacent mantle rock. At divergent boundaries magma forms because of decompression melting. Decompression melting also takes place within a mantle plume.
11.9.6 Volcanic Hazards
Most direct volcanic hazards are related to volcanoes that erupt explosively, especially composite volcanoes. Pyroclastic flows, some as hot as 1000 ˚C, can move at hundreds of km/h and will kill anything in the way. Lahars, volcano-related mudflows, can be large enough to destroy entire towns. Lava flows are also destructive, but tend to move slowly enough to permit people to get to safety. Indirect hazards claim far more lives than direct hazards, and include famine related to volcanically-induced climate cooling.
11.9.7 Monitoring Volcanoes and Predicting Eruptions
Clues that a volcanic eruption might soon occur include earthquakes, a change in the type and amount of gases released, and changes in the shape of the volcano as magma moves within it. Volcanoes are monitored using seismometers to detect earthquakes, volcanic gases are sampled and analyzed, and instruments are used to detect deformation of the volcano. These tools make it possible to assess the hazard posed by a given volcano, and the risk of eruption.
11.9.8 Volcanoes in British Columbia
British Columbia and the Yukon Territory include examples of volcanoes that form as a result of fluid-induced melting along a subduction zone (the Wrangell and Garibaldi volcanic belts) , as a result of decompression where the crust is thinning and stretching (Stikine Volcanic Belt and Wells Gray-Clearwater Volcanic Field), and because of mantle plume activity (Anahim Volcanic Belt).
11.10 Chapter Review Questions
What are the three main tectonic settings for volcanism on Earth?
What is the primary mechanism for partial melting at a convergent plate boundary?
Why are the viscosity and gas content of a magma important in determining the type of volcanic rocks that will be formed when that magma is extruded?
Why do the gases in magma not form gas bubbles when the magma is deep within the crust?
Where and why do pillow lavas form?
What two kinds of volcanic materials make up a composite volcano?
What is a lahar, and why are lahars commonly associated with eruptions of composite volcanoes?
Under what other circumstances might a lahar form?
Why do shield volcanoes have gentle slopes?
Which type of volcanic mountain would last longest: a shield volcano, a cinder cone, or a composite volcano?
Why is weak seismic activity (small earthquakes) typically associated with the early stages of a volcanic eruption?
How can GPS technology be used to help monitor a volcano for activity?
What is the likely geological origin of the Nazko Cone?
What might be the explanation for southwestern B.C. having much less subduction-related volcanism than adjacent Washington and Oregon?
11.11 Answers to Chapter Review Questions
The three main tectonic settings for volcanism are (1) subduction zones at convergent plate boundaries, (2) divergent plate boundaries, and (3) mantle plumes (a.k.a. hot spots).
The primary mechanism for partial melting at a convergent plate boundary is the addition of water to hot mantle rock. The water reduces the melting temperature of the rock (flux melting).
The explosiveness of a volcanic eruption depends on the pressure of the magma. Gases create that pressure, and if the magma is viscous those gases cannot escape easily. Felsic and intermediate magmas tend to have more gas than mafic magmas, and are also more viscous, trapping the gas in.
When magma is deep within the crust the pressure is too high for the gases to bubble out of solution.
Pillow lavas form where mafic lava erupts in water. When the magma oozes out into the water the outside cools first forming a hard skin that maintains the pillow shape.
Composite volcanoes are formed of layers of lava flows and tephra (volcanic fragments ranging from fine ash to blocks and bombs) from explosive eruptions.
A lahar is a mud flow or debris flow on a volcano. Lahars are common on composite volcanoes because they are steeper than shield volcanoes, they typically have ice and snow, and they are not as strong as shield volcanoes.
Some lahars form during an eruption when snow and ice melt quickly, while others may form from heavy rain.
The lava that forms shield volcanoes is typically low viscosity. It can flow easily and also tends to form lava tubes. As a result, it is able to travel a long way from the vent, forming a low broad shield.
Cinder cones erode rapidly because they are mostly piles of tephra. Composite volcanoes are more resistant to weathering and erosion than cinder cones because lava flows help hold together tephra, but composite volcanoes still don’t last as long as shield volcanoes. Shield volcanoes are stronger because they consist more of lava flows than tephra.
Weak seismic activity is associated with all stages of a volcanic eruption. In the early stages magma is moving at depth and pushing rock aside, creating small earthquakes. The flow of magma can also produce special type of seismic response known as a harmonic tremor.
GPS technology is used to determine if there is any slow deformation of the flanks of a volcano related to movement of magma toward the surface.
The Nazko Cone is thought to be related to a mantle plume.
One hypothesis to explain the lower rate of volcanism in British Columbia than in adjacent Washington and Oregon is that the northern part of the Juan de Fuca Plateis not subducting as quickly as the rest of the plate.
11.12 References
Rubin, K. (n.d.) _Mauna Loa Volcano. _Retrieved 23 August 2017. Visit website
Bressan, D (2012). Geology Scene Investigation: Death by Volcanic Fire. Visit website
British Geological Survey (n.d.). Eyjafjallajökull eruption, Iceland | April/May 2010. Visit website_ _
Digital History Project (2011). “Eyewitness Account to Eruption of Mont Pelee Matinique St Pierre Fort de France” By Ellery S. Scott. Visit website_ _
Rosen, J. (2015). Benchmarks: May 8, 1902: The deadly eruption of Mount Pelée. Visit website
U. S. Geological Survey (1997). Pyroclastic flows. Visit website
Kiser, E., Palomeras, I., Levander, A., Zelt, C., Harder, S., Schmandt, B., Hansen, S., Creager, K., & Ulberg, C. (2016).Magma reservoirs from the upper crust to the Moho inferred from high-resolution Vp and Vs models beneath Mount St. Helens, Washington State, USA. _Geology (44)_6, 411-414. DOI: 10.1130/G37591.1
Lin, G, Amelung, F, Lavallee, Y, and Okubo, P. (2014). Seismic evidence for a crustal magma reservoir beneath the upper east rift zone of Kilauea volcano, Hawaii. Geology, 42(3), 187-190. DOI: 10.1130/G35001.1
Regelous, M., Hofmann, A. W., Abouchami, W., & Galer, S. J. G. (2003) Geochemistry of lavas from the Emperor Seamounts, and the geochemical evolution of Hawaiian magmatism from 85 to 42 Ma. Journal of Petrology 44(1), 113-140. DOI: 10.1093/petrology/44.1.113 view PDF
U. S. Geological Survey, Volcano Hazards Program (n.d.). Indian Heaven Volcanic Field visit website
U. S. Geological Survey, Volcano Hazards Program (n.d.). Mount St. Helens: 1980 Cataclysmic Eruption visit website
Geological Survey of Canada (n.d.) Catalog of Canadian Volcanoes: Anahim volcanic belt Visit website
Geological Survey of Canada (n.d.) Catalog of Canadian Volcanoes: Garibaldi volcanic belt: Garibaldi Lake volcanic field Visit website
Skulski, T., Francis, D., & Ludden, J. (1991) Arc-transform magmatism in the Wrangell volcanic belt. _Geology (19)_1, 11-14. doi:10.1130/0091-7613(1991)019<0011:ATMITW>2.3.CO;2
Trop, J. M., Hart, W. K., Snyder, D., & Idleman, B. (2012). Miocene basin development and volcanism along a strike-slip to flat-slab subduction transition: Stratigraphy, geochemistry, and geochronology of the central Wrangell volcanic belt, Yakutat-North America collision zone. _Geosphere (8)_4, 805-834. doi:10.1130/GES00762.1
Volcanoes Canada, Canadian Hazards Information System, Natural Resources Canada (n.d.). Where Are Canada’s Volcanoes? Visit website
Friedrich, W. L., Kromer, B., Friedrich, M., Heinemeier, J., Pfeiffer, T., & Talamo, S. (2006). Santorini Eruption Radiocarbon Dated 1627-1600 B.C. Science (312)5773, 548. doi: 10.1126/science.1125087↩︎