Chapter 17 Glaciation
Adapted from Physical Geology, First University of Saskatchewan Edition (Joyce M. McBeth) and Physical Geology (Steven Earle)
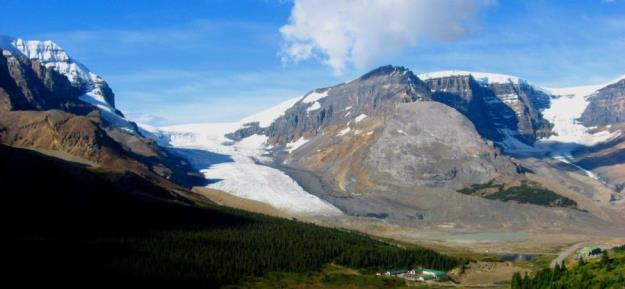_](figures/17-glaciation/figure-17-1.png)
Figure 17.1: Glaciers in the Alberta Rockies: Athabasca Glacier (centre left), Dome Glacier (right), and the Columbia Icefield (visible above both glaciers). The Athabasca Glacier has prominent lateral moraines on both sides. Source: Steven Earle (2015) CC BY 4.0 view source
Learning Objectives
After reading this chapter and answering the review questions at the end, you should be able to:
- Define, draw, and describe the major features of glaciers
- Explain the differences between continental and alpine glaciation
- Summarize how snow and ice accumulate above a glacier’s equilibrium line and are converted to ice
- Explain how basal sliding and internal flow facilitates the movement of ice from the upper part to the lower part of an alpine glacier
- Describe and identify the various landforms related to alpine glacial erosion, including U-shaped valleys, arêtes, cols, horns, hanging valleys, truncated spurs, drumlins, roche moutonnées, glacial grooves, and striae
- Identify various types of glacial lakes, including tarns, finger lakes, moraine lakes, and kettle lakes
- Describe the nature and origin of lodgement till and ablation till
- Describe the nature and origin of glaciofluvial, glaciolacustrine, and glaciomarine sediments
- Describe the timing and extent of Earth’s past glaciations, going as far back as the early Proterozoic
- Describe the important geological events that led up to the Pleistocene glaciations
- Explain how the Milankovitch orbital variations along with positive climate feedback mechanisms may have controlled the timing of the Pleistocene glaciations
A glacier is a long-lasting (decades or more) body of ice that is large enough to move under its own weight. They are at least tens of metres thick and at least hundreds of metres in extent. About 10% of Earth’s land surface is currently covered with glacial ice, and although the vast majority of this is in Antarctica and Greenland, there are many glaciers in Canada, especially in the mountainous parts of BC, Alberta, and the Yukon, and in the far north (Figure 17.1). At various times during the past million years, glacial ice has been much more extensive, covering at least 30% of the Earth’s land surface at times.
Glaciers currently represent the largest repository of fresh water on Earth (~69% of all fresh water). They are highly sensitive to changes in climate, and in recent decades have been melting rapidly worldwide (Figure 17.2). Although some of the larger glacial masses may still last for several centuries, smaller glaciers, including many in western Canada, may be gone within decades. For mountainous regions, glaciers are an important sources of drinking water. Rapid glacial melting is a troubling issue for western Canadians because glacial ice is an important part of the hydrologic cycle in glaciated regions. Irrigation systems in BC, and across Alberta and Saskatchewan, are replenished by meltwater originating from glaciers in the Coast Range and the Rocky Mountains.
_](figures/17-glaciation/figure-17-2.jpg)
Figure 17.2: Example of rapid melting of a glacier over a 63-year period. Muir Glacier, Alaska. Source: NASA Climate 365 Project (n.d.) Public Domain view source
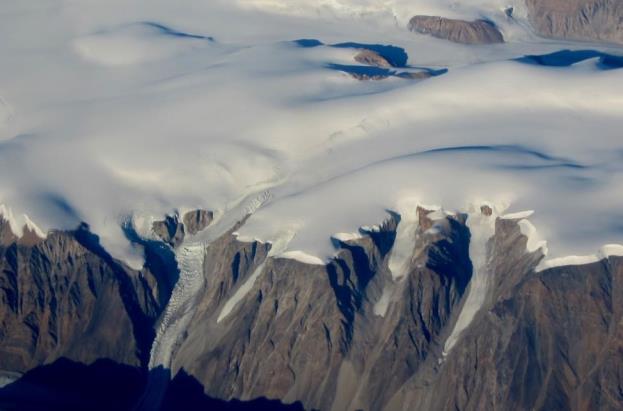_](figures/17-glaciation/figure-17-3.jpg)
Figure 17.3: Part of the continental ice sheet in Greenland, with some outflow alpine glaciers in the foreground. Source: Steven Earle (2015) CC BY 4.0 view source
17.1 Types of Glaciers
There are two main types of glaciers: continental glaciers and alpine glaciers. Latitude, topography, and global and regional climate patterns are important controls on the distribution and size of these glaciers.
17.1.1 Continental Glaciers
Continental glaciers cover vast areas of land. Today, continental glaciers are only present in extreme polar regions: Antarctica and Greenland (Figure 17.3). Historically, continental glaciers also covered large regions of Canada Europe, and Asia, and they are responsible for many distinctive topographic features in these regions (Section 17.2 and 17.3).
Continent glaciers can form and grow when climate conditions in a region cool over extended periods of time. Snow can build up over time in regions that do not warm up seasonally, and if the snow accumulates in vast amounts, it can compact under its own weight and form ice.
Earth’s two current continental glaciers, the Antarctic and Greenland Ice Sheets, comprise about 99% of Earth’s glacial ice, and approximately 68% of Earth’s fresh water. The Antarctic Ice Sheet is vastly larger than the Greenland Ice Sheet (Figure 17.4) and contains about 17 times as much ice. If the entire Antarctic Ice Sheet melted, sea level would rise by about 80 m and most of Earth’s major coastal cities would be submerged.
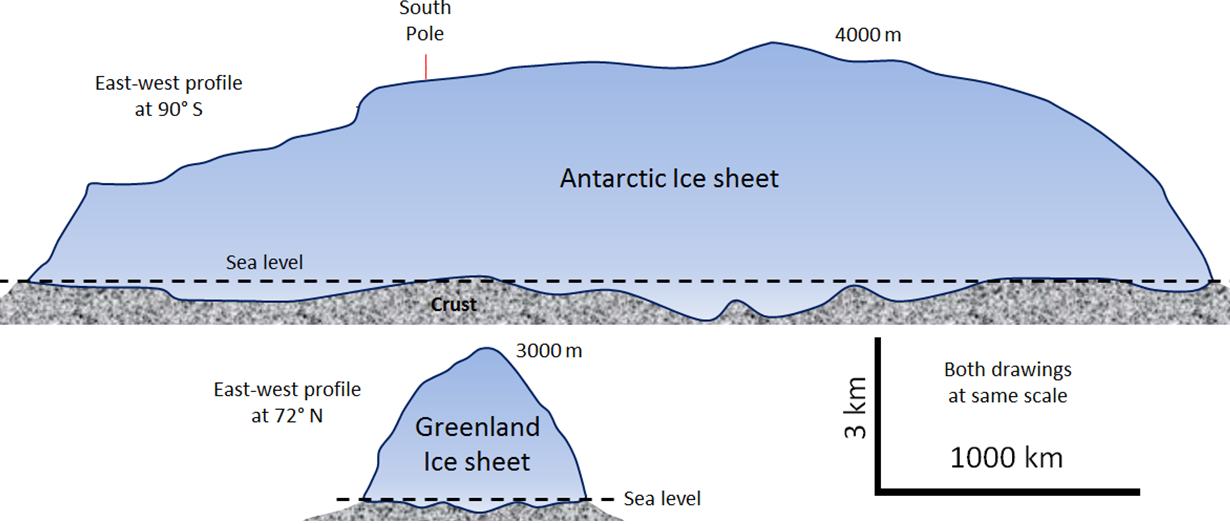_](figures/17-glaciation/figure-17-4.png)
Figure 17.4: Simplified cross-section profiles of the Antarctic and Greenland continental ice sheets. Both ice sheets are drawn to the same scale (exaggerated in the vertical direction). Source: Steven Earle (2015) CC BY 4.0 view source
Continental glaciers generally cover areas that are flat, but the force of gravity still acts on them and causes them to flow. Continental glacier ice flows from the region where it is thickest toward the edges where it is thinner (Figure 17.5). In the central thickest parts, the ice flows almost vertically down toward the base, while at the edges of the glacier, it flows horizontally out toward the margins. In continental glaciers like the Antarctic and Greenland Ice Sheets, the thickest parts (4,000 m and 3,000 m thick, respectively) are the areas where the rate of snowfall, and therefore of ice accumulation, are greatest. In Antarctica, the ice sheet flows out over the ocean, forming ice shelves. Ice shelves can slow the flow of continental glaciers outward. Conversely, if ice shelves break down continental glacier flow can speed up.
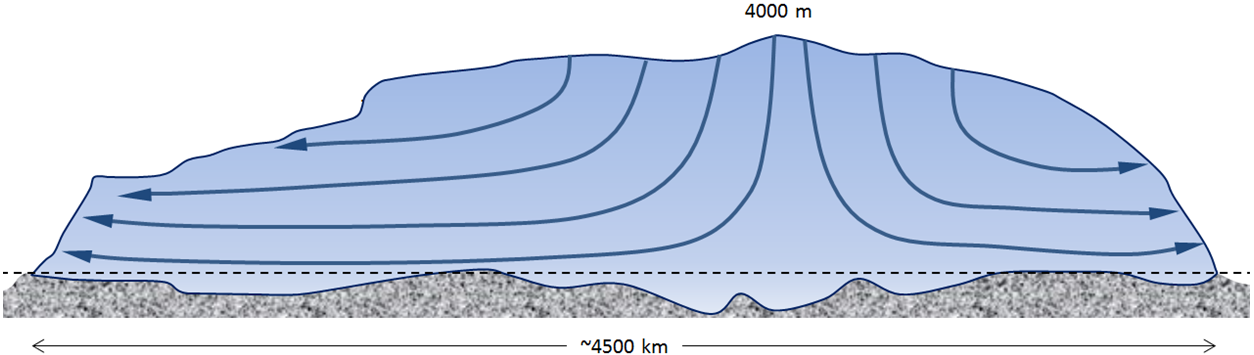_](figures/17-glaciation/figure-17-5.png)
Figure 17.5: Cross-section showing ice-flow in the Antarctic Ice Sheet. Source: Steven Earle (2015) CC BY 4.0 view source
17.1.2 Alpine Glaciers
Alpine glaciers (aka valley glaciers) originate high up in the mountains, mostly in temperate and polar regions (Figure 17.1), but also in tropical regions in high mountains (e.g. in the Andes Mountains of South America).
The flow of alpine glaciers is driven by gravity, and primarily controlled by the slope of the ice surface (Figure 17.6). Alpine glaciers grow due to accumulation of snow over time. In the zone of accumulation, the rate of snowfall is greater than the rate of melting. In other words, not all of the snow that falls each winter melts during the following summer, and the ice surface in the zone of accumulation does not lose its annual accumulation of snow cover over the course of the year. In the zone of ablation, the rate of melting exceeds accumulation. The equilibrium line marks the boundary between the zones of accumulation (above) and ablation (below) (Figure 17.6).
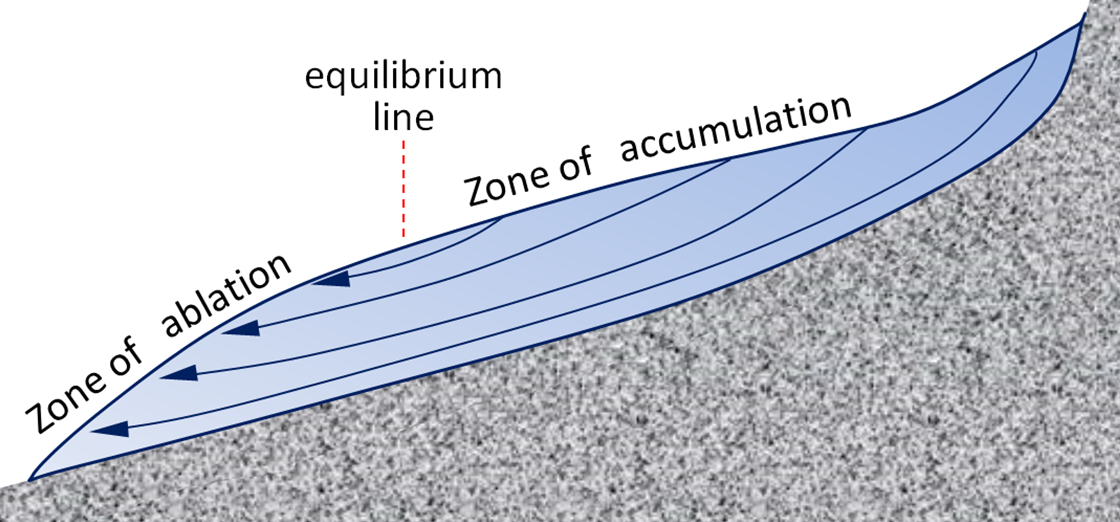_](figures/17-glaciation/figure-17-6.jpg)
Figure 17.6: Schematic diagram illustrating alpine glacier ice-flow. Source: Steven Earle (2015) CC BY 4.0 view source
Above the equilibrium line of a glacier, winter snow will remain even after summer melting, so snow gradually accumulates on the glacier over time. The snow layer from each year is covered and compacted by subsequent snow, and it is gradually compressed and converted to firn (Figure 17.7).
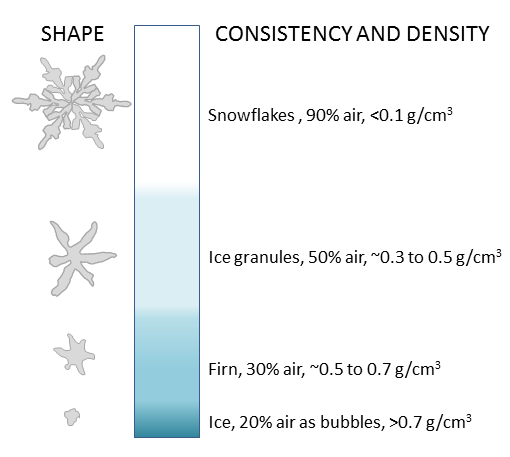_](figures/17-glaciation/figure-17-7.png)
Figure 17.7: Steps in the process of formation of glacial ice from snow, granules, and firn. Source: Steven Earle (2015) CC BY 4.0 view source
Firn is a form of ice that forms when snowflakes lose their delicate shapes and become granules due to compression. With more compression, the granules are squeezed together, and air is forced out. Eventually the granules are “welded” together to create glacial ice (Figure 17.7). Downward percolation and freezing of water from melting contributes to the process of ice formation.
The equilibrium line of a glacier near Whistler, BC, is shown in Figure 17.8 Below this line is the zone of ablation. In the zone of ablation, bare ice is exposed because the previous winter’s snow has all melted. Above this line the ice is still mostly covered with snow from the previous winter.
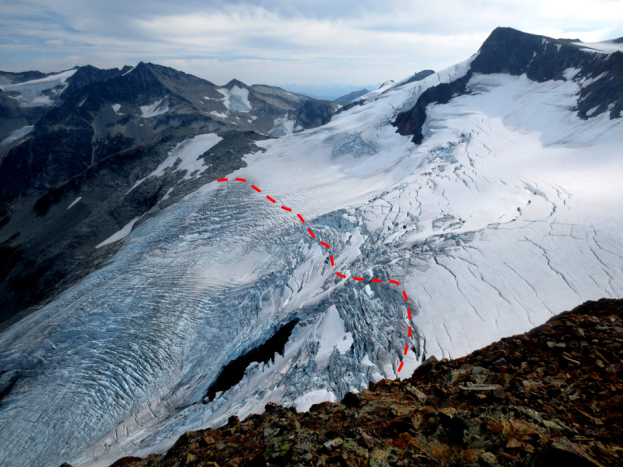_](figures/17-glaciation/figure-17-8.png)
Figure 17.8: The approximate location of the equilibrium line (red) in September 2013 on the Overlord Glacier, near Whistler, B.C. Source: Steven Earle (2015) CC BY 4.0, after Isaac Earle (n.d.) CC BY 4.0 view source
The position of the equilibrium line changes from year to year as a function of the balance between snow accumulation in the winter, and snow and ice melt during the summer. If there is more winter snow and less summer melting, this favours the advance of the equilibrium line down the glacier (and ultimately increases the size of the glacier). Between accumulation and melting, the summer melt matters most to a glacier’s ice budget. Cool summers promote an increase in glacier size, and thus lead to advance of the equilibrium line. Warm summers promote melting, and retreat of the equilibrium line.
Alpine glaciers move because they are heavy, and the force of gravity acts on the ice in the glacier to pull it down the slope of the mountains where they form. The movement of the glacier generates stress in the ice, which is proportional to the slope of the glaciers surface features of the underlying rock surface, and to the depth within the glacier.
As shown in Figure 17.9, the stresses are relatively small near the ice surface but much larger at depth. Stresses are greater in areas where the ice surface is relatively steep.
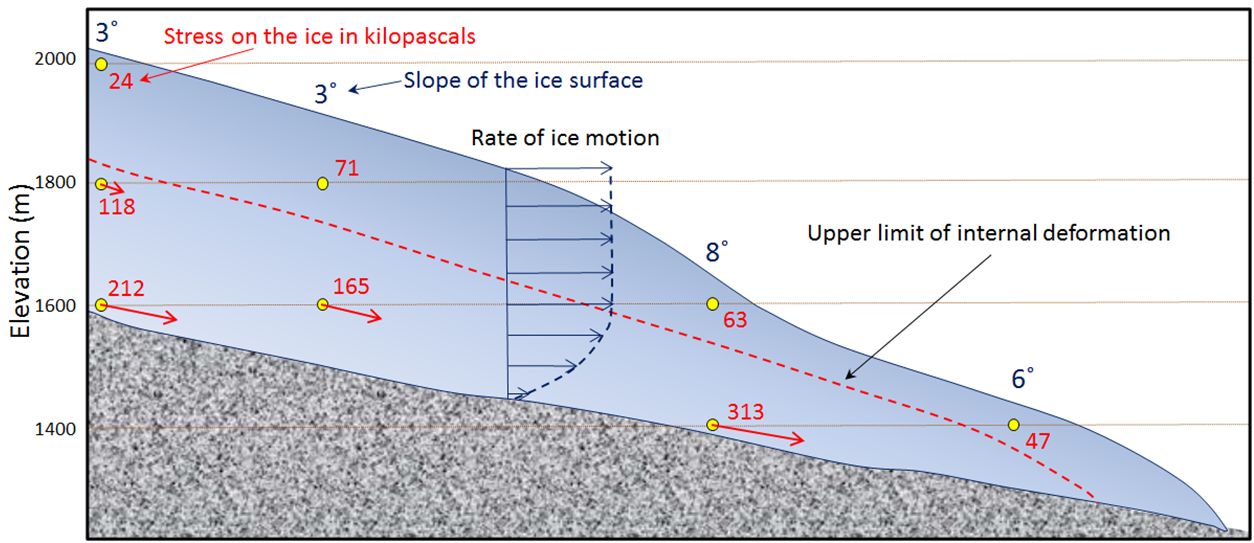_](figures/17-glaciation/figure-17-9.png)
Figure 17.9: Stress within an alpine glacier (red numbers) as determined from the slope of the ice surface and the depth within the ice. The ice will deform and flow where the stress is greater than about 100 kilopascals, and regions with higher rates of deformation are depicted by the red arrows. Any motion of the lower ice will be transmitted to the ice above it, so although the red arrows get shorter toward the top, the ice is still moving (blue arrows in centre of diagram inset illustrate rate of ice motion). The upper ice (above the red dashed line) does not flow plastically, but it is carried along with the lower ice. Source: Joyce McBeth (2018) CC BY 4.0, modified after Steven Earle (2016) CC BY 4.0 view source
Like rock, ice behaves in a brittle fashion under low pressure conditions (shallow depths in the glacier), and plastically at higher pressures (deeper in the glacier). Stress also affects how ice deforms; at high stress ice will either break or deform plastically (ductile deformation) depending on the pressure conditions. Under brittle deformation conditions (low pressures, shallow depths in the glacier), stress is released when the ice cracks, so does not build up to high values. Within the upper 50 - 100 m of ice (above the dashed red line, in Figure 17.9), flow is brittle: the ice is rigid and will crack in response to stress. Under ductile deformation conditions (higher pressures deeper in the glacier), stress can accumulate, and the ice will flow plastically in response to that stress. Ice deforms plastically if deeper than about 100 m in the glacier, and in this region stress levels can accumulate to high values (100 kilopascals or greater, Figure 17.9).
When the lower ice of a glacier flows, it moves the upper ice along with it. It may seem from the stress patterns (red numbers and arrows in Figure 17.9) that the lower ice moves more or faster than the upper ice, but this is not the case. The lower ice deforms (flows) and the upper part is carried along and deforms through brittle deformation if subjected to sufficient stress. The upper part of the glacier moves faster than the base of the glacier because there is friction between the base of the glacier and the surface beneath it that slows the movement of the ice at the base.
The plastic lower ice of a glacier can flow over irregularities in the rocks under the glacier. However, the upper rigid ice cannot flow in this way, and because it is being carried along by the lower ice, it tends to crack in locations when the lower ice flows over changes in the topography below the glacier. This leads to formation of crevasses in areas where the rate of flow of the deeper, plastic ice is changing. In the area shown in Figure 17.10, for example, the glacier is accelerating over the steep terrain, and the rigid surface ice cracks to release stress that accumulates due to the change in velocity and tension in the ice.
_](figures/17-glaciation/figure-17-10.jpg)
Figure 17.10: Crevasses in a glacier in Mount Cook National Park, New Zealand. _Source: Bernard Spragg (2008) CC0 1.0 __view source_
In addition to deformation, another important aspect of glacier flow is basal sliding, which is sliding movement between the base of the glacier and the underlying material. The base of a glacier can be cold (below the freezing point of water) or warm (above the freezing point). If it is warm, a film of water can form between the ice and the material underneath, and the ice will be able to slide over this surface (Figure 17.11, left). If the base is cold, the ice will be frozen to the material underneath and it will be stuck — unable to slide along its base. In this case, all the movement of the ice will be by internal flow.
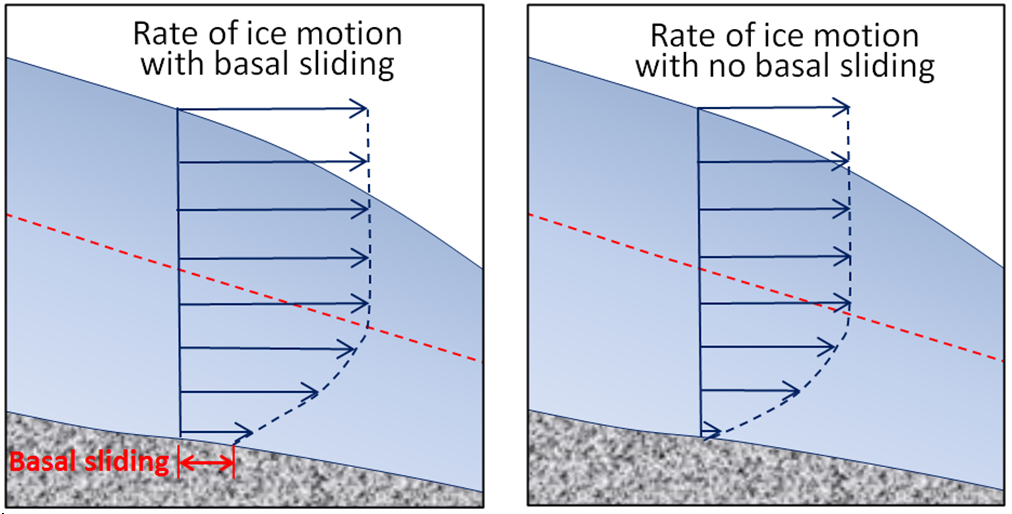_](figures/17-glaciation/figure-17-11.png)
Figure 17.11: Differences in glacial ice motion with basal sliding (left) and without basal sliding (right). The dashed red line indicates the upper limit of plastic internal flow. Source: Steven Earle (2016) CC BY 4.0 view source
There are several factors that can influence warming of the ice and basal flow at the base of an alpine glacier. Friction between the base of the glacier and the surface underneath generates heat and can lead to melting of the ice at the base of the glacier. Rainwater and meltwater from upper regions of the glacier can percolate down and transfer heat to warm the base of the glacier and enhance basal sliding, particularly in warmer seasons. Geothermal heat from below also contributes to melting at the base of glaciers in regions with high heat flow due to volcanic activity.
Another factor that controls the temperature at the base of a glacier is the thickness of the ice. The force of gravity acting on thicker ice can enhance friction and melting at the base. Ice is also a good insulator so can prevent accumulated heat from escaping. The leading edge of an alpine glacier is typically relatively thin (see Figure 17.9), so it is common for this part to be frozen to its base while the rest of the glacier is still sliding. Since the leading edge of the glacier is frozen to the ground, and the rest of the glacier behind continues to slide forward, this causes the trailing ice to be pushed (or thrust) over top of the leading edge, forming thrust faults in the ice (Figure 17.12).
_](figures/17-glaciation/figure-17-12.jpg)
Figure 17.12: Thrust faults at the leading edge of the Byron Glacier, Portage Lake, Alaska, USA. The dark stripes are sediments that were entrained in the base of the glacier ice and transported up along the thrust faults. Source: Cindy Zackowitz (2011) CC BY-NC 2.0 view source
Just as the base of a glacier moves slower than the surface, the edges, which are more affected by friction along the channel walls, also move slower. If we were to place a series of markers across an alpine glacier and come back a year later, we would see that the ones in the middle had moved further forward than the ones near the edges (Figure 17.13).
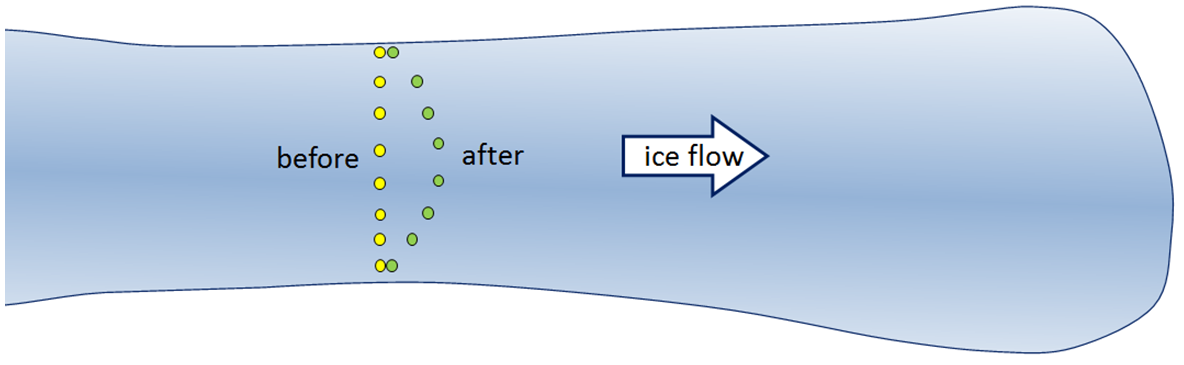](figures/17-glaciation/figure-17-13.png)
Figure 17.13: Markers on an alpine glacier move forward at different rates over a period of time. Source: Steven Earle (2016) CC BY 4.0 view source
Alpine glacial ice continuously moves down the slope of the ice in response to gravity, but it may not appear to be moving because the front edge of a glacier is also continuously losing volume. It either melts or, if they glacier terminates at a lake or ocean, the front edge will calve into the water (break off pieces of the front edge of the glacier that become icebergs). If the rate of forward motion of the glacier is faster than the rate of ablation (melting), the leading edge of the glacier advances (moves forward). If the rate of forward motion is about the same as the rate of ablation, the leading edge remains stationary, and if the rate of forward motion is slower than the rate of ablation, the leading-edge retreats (moves backward).
Calving of icebergs is an important process for glaciers that terminate in lakes or oceans. An example of such a glacier is the Berg Glacier on Mt. Robson (Figure 17.14), which sheds small icebergs into Berg Lake. The Berg Glacier also lose mass by melting, evaporation, and sublimation.
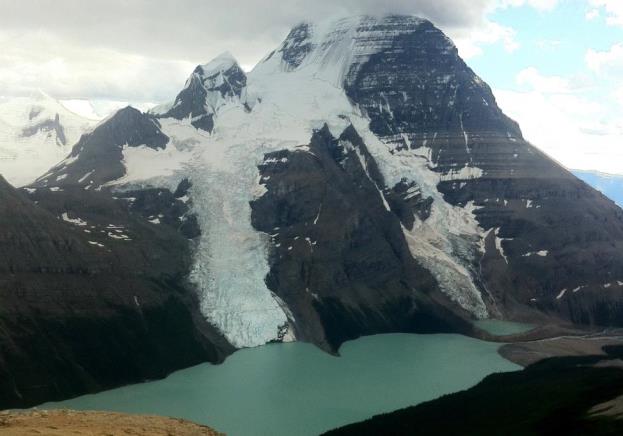](figures/17-glaciation/figure-17-14.jpg)
Figure 17.14: Mt. Robson, the tallest peak in the Canadian Rockies, hosts the Berg Glacier (centre), and Berg Lake. Although there were no icebergs visible when this photo was taken, the Berg Glacier loses mass by shedding icebergs into Berg Lake. Source: Steven Earle (2015) CC BY 4.0 view source
Exercise: Ice Advance and Retreat
These diagrams in Figure 17.15 represent a glacier with markers placed on its surface to determine the rate of ice motion over a one-year period. The ice is flowing from left to right.
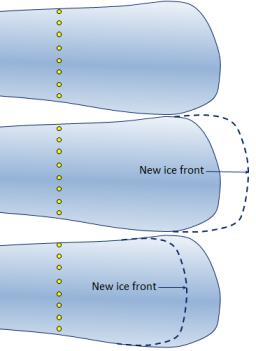_](figures/17-glaciation/figure-17-15.jpg)
Figure 17.15: Glacier with markers to show rate of motion. Source: Steven Earle (2015) CC BY 4.0 view source
In the middle diagram, the leading edge of the glacier has advanced. Draw in the current position of the markers.
In the lower diagram, the leading edge of the glacier has retreated. Draw in the current position of the markers.
17.2 Glacial Erosion
Glaciers are effective agents of erosion, especially in situations where the base of the glacier is not frozen to the underlying material and can therefore slide over the bedrock or other sediment. The ice itself is not particularly effective at erosion because it is relatively soft (Mohs hardness 1.5 at 0°C). Glacial erosion is primarily driven by abrasion of the underlying rocks by rock fragments embedded within the ice. These rocks are pushed down onto the underlying surfaces by the ice, and because they are hard they can gouge and grind down the materials beneath the glacier. An analogy for these processes is to compare the effect of a regular piece of paper being rubbed against a wooden surface (“ice eroding rock”) to rubbing a piece of sandpaper over the same surface (“ice with embedded rocks eroding rock”). The results of glacial erosion are different in areas with continental glaciation versus alpine glaciation.
17.2.1 Continental Glacial Erosion Features
Continental glaciation tends to produce relatively flat bedrock surfaces, especially where the rock beneath is uniform in strength. In areas where there are differences in the strength of rocks, a glacier tends to erode the softer and weaker rock more effectively than the harder and stronger rock. Much of central and eastern Canada, which was completely covered by the huge Laurentide Ice Sheet at various times during the Pleistocene Epoch, has been eroded to a relatively flat surface. Glacial deposits have created distinctive topographic features on the landscapes in these regions — such as drumlins, eskers, and moraines (Figure 17.16). These continental glacial features are deposits of glacial materials and are described further in Section 17.3.
_](figures/17-glaciation/figure-17-16.png)
Figure 17.16: Landscape features associated with continental glaciation Source: Luis María Benítez (2005) CC BY 4.0 view source
In areas of continental glaciation, the lithosphere is depressed by the weight of glacial ice that is up to 4,000 m thick. Basins formed along the edges of continental glaciers; for example, basins formed around the edges of the Laurentide Ice Sheet that once covered much of Canada (Section 17.4). These basins filled with glacial meltwater, and layers of sediments. Many such lakes, some of them huge, existed at various times along the southern edge of the Laurentide Ice Sheet.
One example of these lakes was Glacial Lake Missoula, which formed within Idaho and Montana, just south of the BC border with the United States. During the latter part of the last glaciation (30 ka to 15 ka), the ice holding back Lake Missoula retreated enough to allow some of the lake water to escape, which escalated into a voluminous and rapid outflow (over days to weeks). During this outflow, most of the lake drained into the Columbia River valley and flowed to the Pacific Ocean. It is estimated that this type of catastrophic outflow happened at least 25 times during this period, and in many cases, the rate of outflow was equivalent to the discharge of all of Earth’s current rivers combined.
17.2.2 Alpine Glacial Erosion Features
Alpine glaciers produce very different topography than continental glaciers. Alpine glaciers produce wide valleys with relatively flat bottoms and steep sides due to the erosion that occurs at the base and edges of the glaciers. These are known as U-shaped valleys (Figure 17.17). In contrast, unglaciated river valleys generally have a V shape.
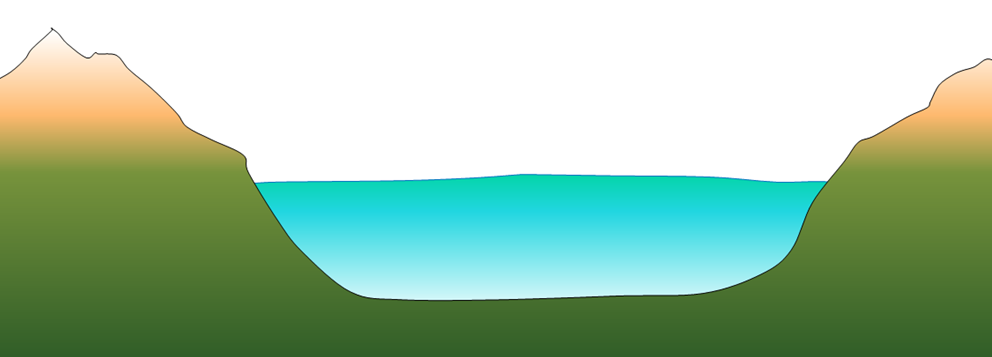](figures/17-glaciation/figure-17-17.png)
Figure 17.17: A depiction of a U-shaped valley occupied by a large glacier. Source: Steven Earle (2016) CC BY 4.0 view source
In coastal regions where the bottom of the valley is filled with water, the U-shaped valleys are called__ fjords__. The coastal mountains of BC have many fine examples of U-shaped valleys and fjords. For example, Howe Sound is a fjord that was once occupied by a large glacier. Howe Sound and most of its tributary valleys have pronounced U-shaped profiles due to glaciation (Figure 17.18).
_](figures/17-glaciation/figure-17-18.jpg)
Figure 17.18: The view down the U-shaped valley of Mill Creek valley toward the U-shaped valley of Howe Sound, with the village of Britannia on the opposite side. Source: Keefer4 (2005) CC BY-SA 2.5 view source
Several other topographic features derived from alpine glacial erosion are found in U-shaped valleys and their tributary valleys (Figure 17.19). Arêtes are sharp ridges formed between U-shaped glacial valleys. Cols are low points (saddles) along arêtes; they form passes (high points) between glacial valleys__. Horns__ are steep peaks that have been eroded by glaciers and freeze-thaw activity on three or more sides. Cirques are bowl-shaped basins that form at the head of a glacial valley, and tarns are lakes that form when cirques are flooded. Hanging valleys form when U-shaped valleys of tributary glaciers connect with a larger U-shaped valley; the tributary valley hangs above the main valley because the larger main-valley glacier is eroded more deeply into the terrain. Truncated spurs (aka “spurs”) are features at the ends of arêtes where the rock is eroded into steep triangle-shaped cliffs by the glacier in the main valley.
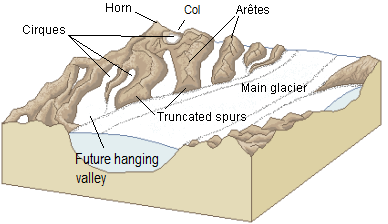. Modified after Luis María Benítez (2005) CC0 1.0 [view source](https://commons.wikimedia.org/wiki/File:Glacial_landscape_LMB.png)_](figures/17-glaciation/figure-17-19.png)
Figure 17.19: A diagram of some of the important alpine-glaciation erosion features. Source: Steven Earle (2015) view source. Modified after Luis María Benítez (2005) CC0 1.0 view source
Figure 17.20 shows examples of these features in the Swiss Alps. The area in the image was intensely glaciated during the past glacial maximum and still contains glaciers. The large U-shaped valley in the lower right was occupied by glacial ice historically, and all of the other glaciers shown here were longer and much thicker than they are now. But even at the peak of the Pleistocene glaciation, some of the higher peaks and ridges in this image would have been exposed and not directly affected by glacial erosion. A peak that extends above the surrounding glacier is called a nunatak. In these areas, and in the areas above the glaciers in the image today, most of the erosion is linked to freeze-thaw action.
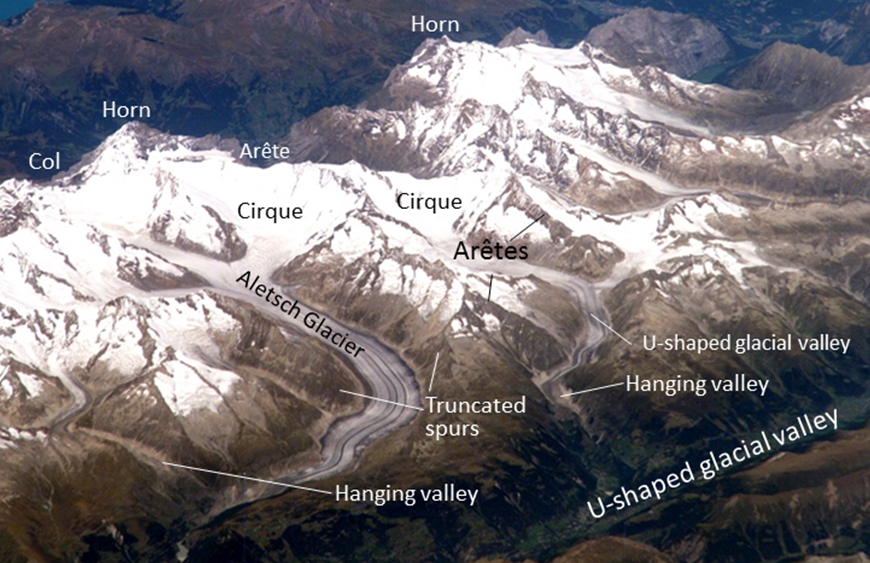. Modified after NASA Earth Observatory (n.d.) Public Domain [view source](https://earthobservatory.nasa.gov/IOTD/view.php?id=7195)_](figures/17-glaciation/figure-17-20.png)
Figure 17.20: A view of the Swiss Alps from the International Space Station, taken in 2006. The region shown is in the area of the Aletsch Glacier. The prominent peaks labelled “Horn” are the famous mountain peaks the Eiger (left) and Wetterhorn (right). A variety of alpine glacial erosion features are labelled. Source: Steven Earle (2016) view source. Modified after NASA Earth Observatory (n.d.) Public Domain view source
A roche moutonnée is aglacial erosion feature that forms when a glacier moves over an outcrop of bedrock. Roche moutonn__é__es consist of a hill of rock, often with a smooth, often low angle slope on one side, and a steeper and jagged slope on the other side. The side that is smooth and relatively low angle is the side the glacier was flowing from, and the a steep and sometimes jagged side is the direction the ice was moving (Figure 17.21, left).
](figures/17-glaciation/figure-17-21.jpg)
Figure 17.21: Roche moutonn__é__e near Myot Hill, Scotland. Source: Chris Upson (2006) CC BY-SA 2.0 view source
Glacial grooves (tens of centimetres to metres wide) and glacial striae (millimetres to centimetres wide) are created by the erosion caused by fragments of rock embedded in the ice at the base of a glacier (Figure 17.22, left and right). Glacial striae are very common on rock surfaces eroded by both alpine and continental glaciers. Glacial polish occurs when the abrasion of the rock by the glacier renders the rock so smooth it reflects light.
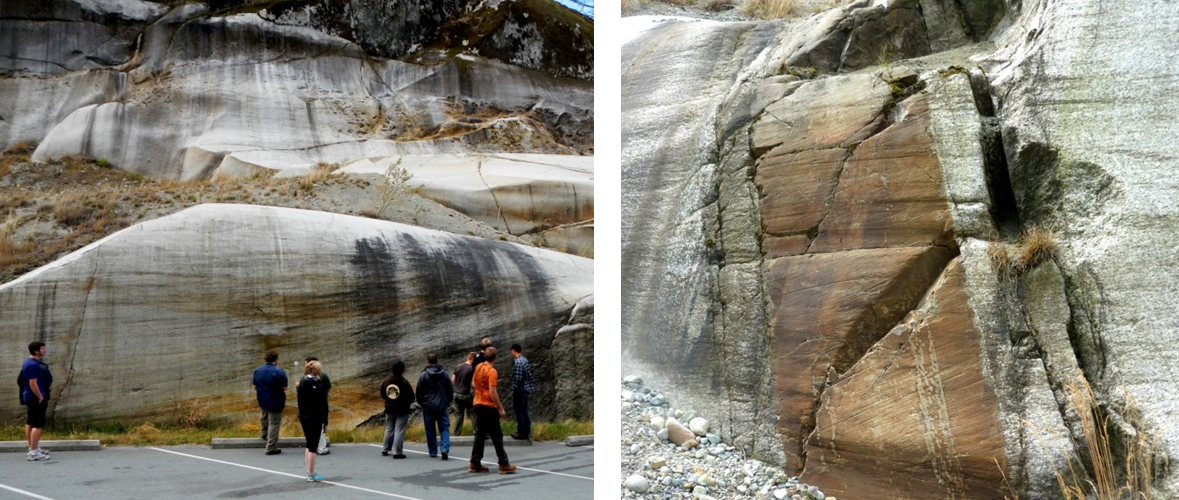_](figures/17-glaciation/figure-17-22.png)
Figure 17.22: Examples of glacial striae from near Squamish, BC. Ice flow was from right to left in both images. Source: Steven Earle (2016) CC BY 4.0 view source
Lakes are common features in glacial environments. A lake that is confined to a glacial cirque is known as a tarn (Figure 17.23). Tarns are common in areas of alpine glaciation because the ice that forms a cirque typically carves out a depression in bedrock that can then fill with water. Moraines, which are linear deposits of glacial sediments (till) left by the glacier along its edges, can form a dam at the end of a tarn. Often, a series of moraines will form as glaciers recede. These can act as water dams, and result in strings of lakes called rock basin lakes or paternoster lakes.
_](figures/17-glaciation/figure-17-23.jpg)
Figure 17.23: Lower Thornton Lake, a tarn, in the Northern Cascades National Park, Washington. Source: Jeff Pang (2007) CC BY 2.0 view source
A lake that occupies a glacial valley is known as a finger lake. In some cases, a finger lake is confined by a dam formed by an end moraine, in which case it may be called a moraine lake (Figure 17.24). Another type of glacial lake is a kettle lake. These are discussed in section 17.4 in the context of glacial deposits.
_](figures/17-glaciation/figure-17-24.jpg)
Figure 17.24: Peyto Lake in the Alberta Rockies, is both a finger lake and a moraine lake, as it is flooding a glacial valley, and is dammed by an end moraine at right. Source: Jeff Hollet (2016) Public Domain view source
Exercise: Identifying Glacial Erosion Features
Figure 17.25 is a photo of Mt. Assiniboine in the BC Rocky Mountains. What are the features at locations a through e? Look for one of each of the following: a horn, an arête, a truncated spur, a cirque, and a col. Try to identify some of the numerous other arêtes in this view, as well as another horn.
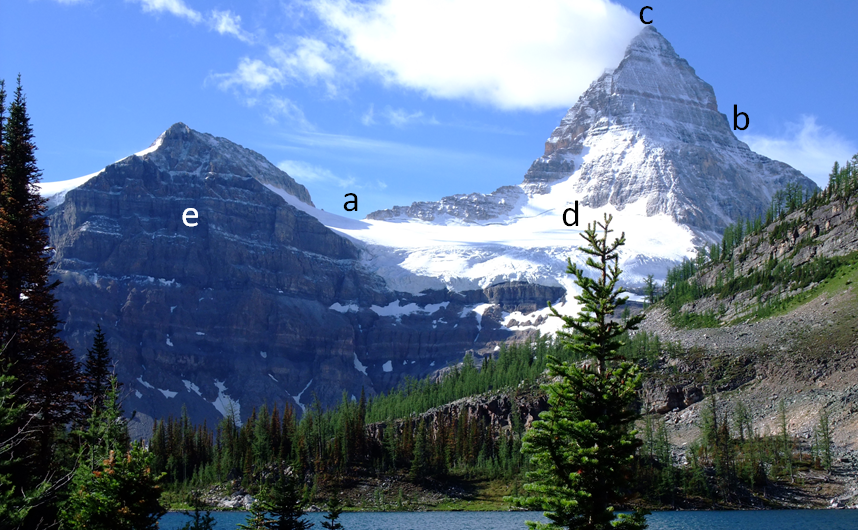. Modified after Kurt Stegmüller (2008) CC BY 3.0 [view source](https://commons.wikimedia.org/wiki/File:Mount_Assiniboine_Sunburst_Lake.jpg)_](figures/17-glaciation/figure-17-25.png)
Figure 17.25: Glacial erosion features of Mt. Assiniboine. Source: Steven Earle (2015) CC BY 4.0 view source. Modified after Kurt Stegmüller (2008) CC BY 3.0 view source
17.3 Glacial Deposits
Sediments transported and deposited during glaciations are abundant throughout Canada. They are important sources of aggregate for construction materials (sand, gravel), and are also important groundwater reservoirs. Because they are almost all unconsolidated, they have significant implications for slope stability and mass wasting.
Figure 17.26 illustrates some of the ways that sediments are transported and deposited by alpine glaciers. The Bering Glacier is the largest glacier in North America, and although most of it is in Alaska, it flows from an icefield that extends into the southwestern Yukon Territory. The surface of the ice is partially, or in some cases completely, covered with rocky debris that has fallen onto the glacier from surrounding steep rock faces. There are muddy rivers issuing from the glacier in several locations, depositing sediment on land, into Vitus Lake, and directly into the ocean. Icebergs are portions of the glacier that have broken off and float away in a lake or ocean. Icebergs are laden with glacial sediments, which are released and deposited as the icebergs melt. Also, not visible in this view, there are sediments being moved along within and beneath the glacier itself.
_](figures/17-glaciation/figure-17-26.jpg)
Figure 17.26: Part of the Bering Glacier in southeast Alaska, the largest glacier in North America. It is about 14 km in width in the centre of this view. Source: Roger Simmon, Landsat 7 Science Team, NASA (2002) Public Domain view source
Sediments are formed and transported in several ways in glacial environments (Figure 17.27). There are many different kinds of glacial sediments, which are generally classified by whether they are transported on, within, or beneath the glacial ice.
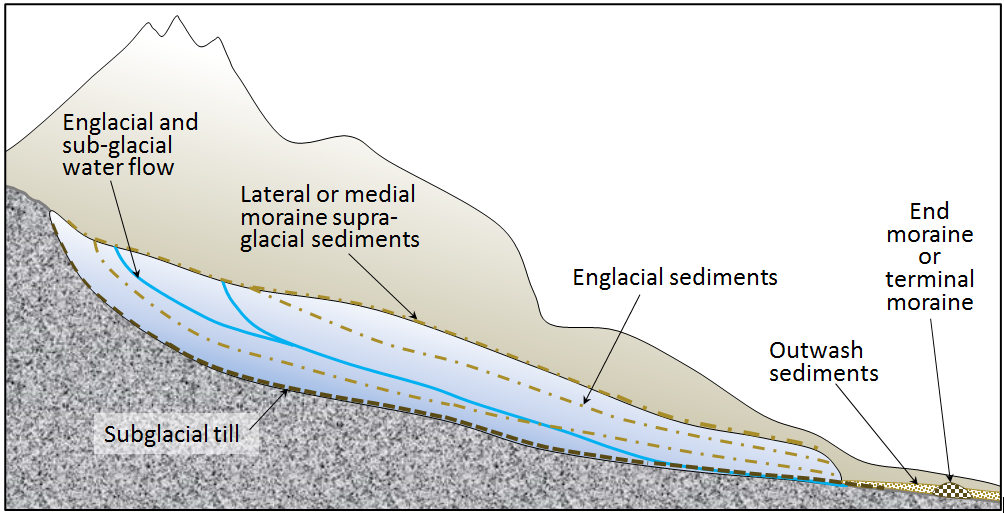_](figures/17-glaciation/figure-17-27.png)
Figure 17.27: A depiction of the various types of sediments associated with the Bering Glacier. The glacier is shown in cross-section. Source: Steven Earle (2016) CC BY 4.0 view source
Supraglacial (on top of the ice) and englacial (within the ice) sediments are released from the melting front of a stationary glacier. These sediments can form a ridge of unsorted sediments called an end moraine. The end moraine from the furthest advance of a glacier is called a terminal moraine. The general name for any sediments transported and deposited by glacial ice is till.
__Subglacial __sediment (e.g., lodgement till) is material that has been eroded from the rock underlying the glacier by the ice and then transported by the ice. It has a wide range of grain sizes, including a relatively high proportion of silt and clay. The larger clasts (pebbles to boulders in size) tend to become partly rounded by abrasion. When a glacier eventually melts, the lodgement till is exposed as a sheet of well-compacted sediment ranging from several centimetres to many metres in thickness. Lodgement till is normally poorly sorted and does not contain bedding features like a lake or stream sediment (Figure 17.28).
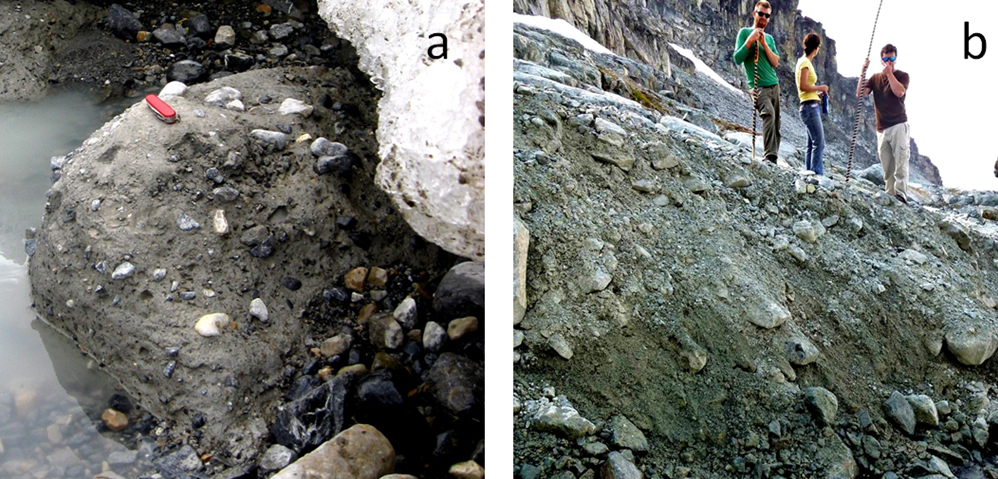](figures/17-glaciation/figure-17-28.png)
Figure 17.28: Examples of glacial till: a: lodgement till from the front of the Athabasca Glacier, Alberta; b: ablation till at the Horstman Glacier, Blackcomb Mountain, BC. Source: Steven Earle (2016) CC BY 4.0 view source
Supraglacial sediments are primarily derived from freeze-thaw eroded material that has fallen onto the ice from rocky slopes above. These sediments form lateral moraines (moraine deposits along the edges of the glacier, see Figure 17.1 for an example). Where two glaciers meet, the sediments form medial moraines (medial moraines are visible in Figure 17.20 and Figure 17.26.) Most of this material is deposited on the ground when the ice melts. This is called ablation till, a mixture of fine and coarse angular rock fragments, with much less sand, silt, and clay than lodgement till (Figure 17.28). When supraglacial sediments become incorporated into the body of the glacier, they are known as englacial sediments (Figure 17.27).
Water flows on the surface, within, and at the base of a glacier, even in cold areas and even when the glacier is advancing. Depending upon its velocity, this water is able to transport sediments of various sizes, and discharges most of these sediments out of the lower end of the glacier, where they are deposited as outwash sediments. These sediments accumulate in a wide range of environments in the proglacial region (the area in front of a glacier). Most of the sediments accumulate in fluvial environments, but some are deposited in lacustrine and marine environments. Glaciofluvial sediments are similar to sediments deposited in normal fluvial environments, but are glacially-derived sediments, and are thus dominated by silt, sand, and gravel. The grains tend to be moderately well rounded and sorted, and the sediments have similar sedimentary structures (e.g., bedding, cross-bedding, clast imbrication [overlapping]) to those formed by non-glacial streams (Figure 17.29).
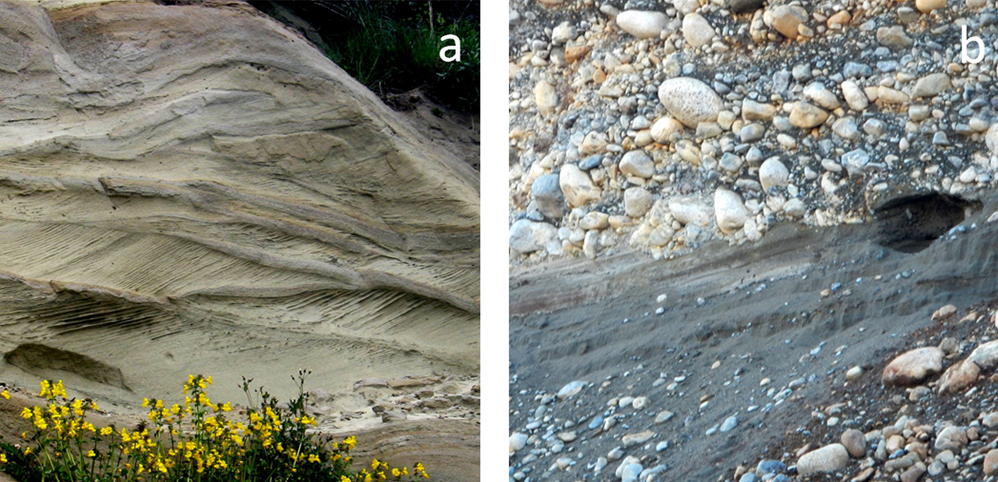](figures/17-glaciation/figure-17-29.png)
Figure 17.29: Examples of glaciofluvial sediments: a: glaciofluvial cross-bedded sand of the Quadra Sand Formation at Comox, BC.; b: glaciofluvial gravel and sand, Nanaimo, BC. Source: Steven Earle (2016) CC BY 4.0 view source
A large proglacial plain of sediment is called a sandur (aka outwash plain)__, __and within this area, glaciofluvial deposits can be tens of metres thick. In situations where a glacier is receding, a block of ice might become separated from the main ice sheet and become buried in glaciofluvial sediments. When the ice block eventually melts, a depression forms, known as a kettle, and if this fills with water, it is known as a kettle lake (Figure 17.30, 17.32). Kettle lakes are also known as pothole lakes or prairie potholes.
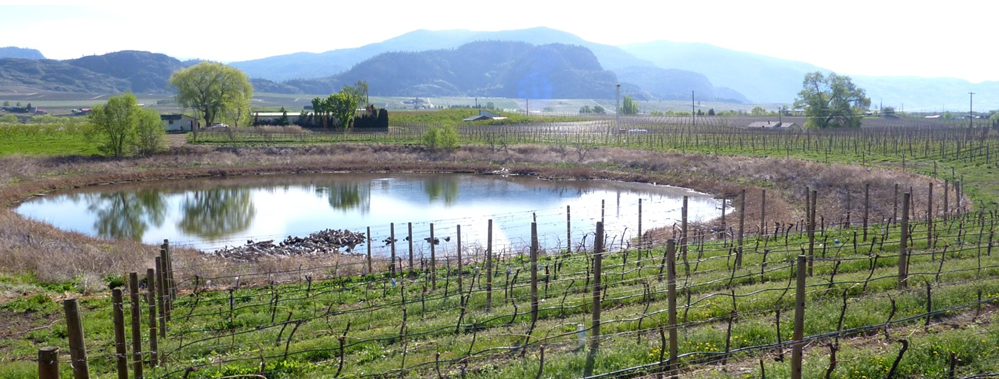](figures/17-glaciation/figure-17-30.png)
Figure 17.30: A kettle lake amid vineyards and orchards in the Osoyoos area of BC. Source: Steven Earle (2015) CC BY 4.0 view source
A supraglacial, englacial, or subglacial stream will create its own channel within the ice, and sediments that are being transported and deposited by the stream will build up within that channel. When the ice melts, the sediment will be deposited upon the underlying ground surface to form a long sinuous ridge known as an esker. Eskers are most common in areas of continental glaciation. They can be several metres high, tens of metres wide, and tens of kilometres long (Figure 17.31). Eskers are commonly comprised of well-sorted sands.
_](figures/17-glaciation/figure-17-31.jpg)
Figure 17.31: Part of an esker that formed beneath the Laurentide Ice Sheet in northern Canada. Source: Gord McKenna (1986) CC BY-NC-ND 2.0 view source
Drumlins are elongated, oval shaped ridges of englacial to subglacial sediments that form at the base of continental glaciers. They are often tens of metres high and hundreds of metres long, and often occur in clusters (“fields”) of tens to hundreds of drumlins (Figure 17.32). As the sediments are deposited, the glacier molds the drumlins’ shapes as the glacier moves over and around them. The long axis of a drumlin is aligned with the direction that the ice moved when the drumlin was deposited.
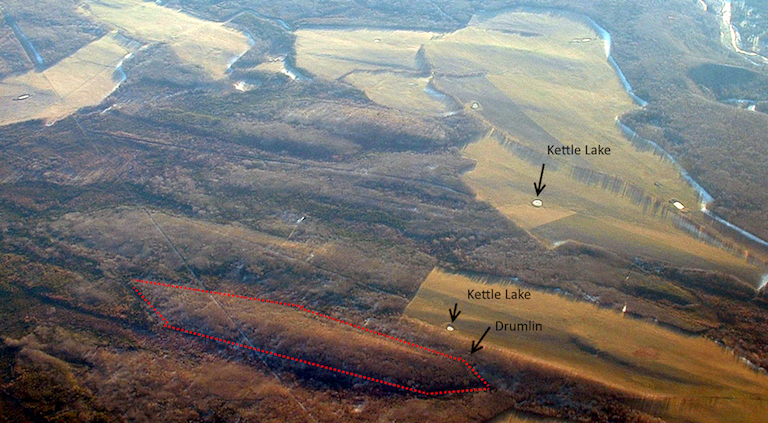
Figure 17.32: Drumlins and kettle lakes viewed from the air near Fort St John, BC. There are numerous drumlins in the image; one is outlined in red. Can you spot the others? Note the alignment of the long axes of the drumlins. Source: Joyce McBeth (2002) CC-BY 4.0. Click the image for a higher resolution version.
Glacial outwash streams commonly flow into proglacial lakes (lakes in front of glaciers) where glaciolacustrine sediments are deposited. These are dominated by silt- and clay-sized particles and are typically laminated (finely layered) on the millimetre scale. In some cases, varves develop. Varves are a series of beds with distinctive summer and winter layers: relatively coarse in the summer when melt discharge is high, and finer in the winter, when discharge is low. Icebergs are common in proglacial lakes, and most of them contain englacial sediments of various sizes. As the icebergs melt, the released clasts sink to the bottom and are incorporated into the glaciolacustrine layers as drop stones (Figure 17.33a). The processes that occur in proglacial lakes can also take place where a glacier terminates in the ocean. The sediments deposited there are called glaciomarine sediments (Figure 17.33b).
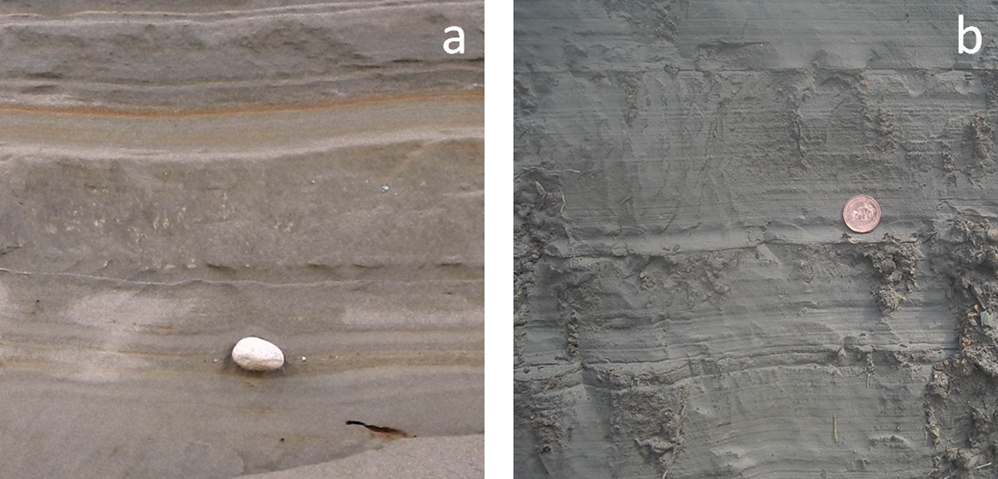](figures/17-glaciation/figure-17-33.png)
Figure 17.33: Examples of glaciolacustrine and glaciomarine sedimentary structures. a: varved glaciolacustrine sediments containing a drop stone, Nanaimo, BC.; and b: a laminated glaciomarine sediment, Englishman River, BC. Source: Steven Earle (2016) CC BY 4.0 view source
Exercise: Identifying Glacial Depositional Environments
Refer to the photo of the Bering Glacier in Alaska shown in Figure 17.26. Glacial sediments of many different types are being deposited throughout the region depicted in this photo.
Identify where you would expect to fine the following: (a) glaciofluvial sand (b) lodgement till (c) glaciolacustrine clay with drop stones (d) ablation till (e) glaciomarine silt and clay
17.4 Glaciations over Earth’s History
We are currently living in the middle of a glacial period, though it is less intense now than it was 20,000 years ago. This is not the only period of glaciation in Earth’s history; there have been many in the distant past (Figure 17.34). In general, however, over the course of Earth’s history the Earth’s surface has been warm and ice-free for longer periods than it has been cold and glaciated.
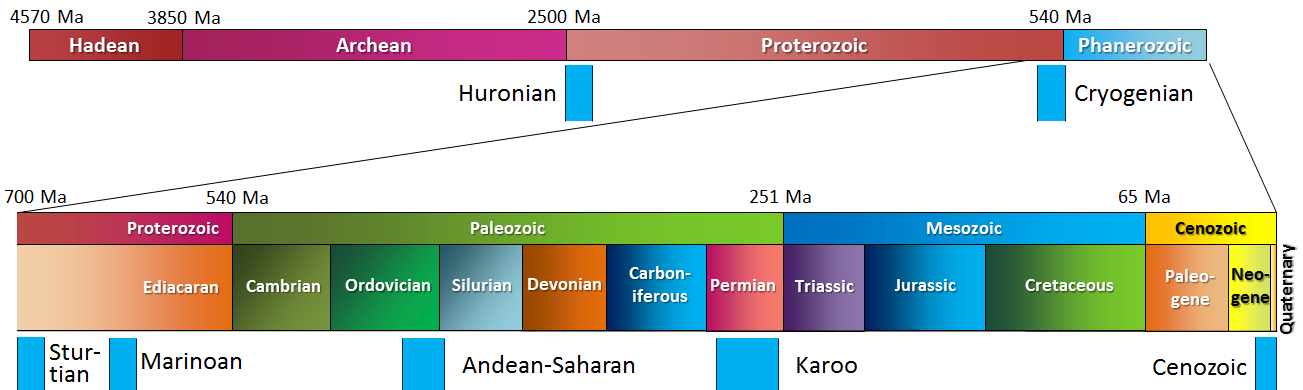](figures/17-glaciation/figure-17-34.png)
Figure 17.34: The record of major past glaciations during Earth’s history. Source: Steven Earle (2015) CC BY 4.0 view source
Pre-Cenozoic Glaciations
The oldest known glacial period is the Huronian. Based on evidence of glacial deposits from the area around Lake Huron in Ontario and elsewhere, it is evident that the Huronian Glaciation lasted from approximately 2.4 to 2.1 Ga. Because rocks of that age are rare, we do not know much about the intensity or global extent of this glaciation.
Late in the Proterozoic, for reasons that are not fully understood, the climate cooled dramatically, and Earth had the most intense time of glaciation it has ever experienced. The glaciations of the Cryogenian Period (cryo is Latin for icy cold) are also known as the “Snowball Earth” glaciations. Scientists have hypothesized that the entire planet was frozen at this time — even in equatorial regions — with ice on the oceans up to 1 km thick. A visitor to our planet at that time would not have found it habitable, although life still survived in the oceans.
There were two main glacial periods within the Cryogenian, each lasting for about 20 million years: the Sturtian at around 700 Ma and the Marinoan at 650 Ma. There is also evidence of some shorter glaciations both before and after these longer periods of glaciation. The end of the Cryogenian glaciations coincides with the evolution of relatively large and complex life forms on Earth. This started during the Ediacaran Period, and then continued with the so-called explosion of life forms in the Cambrian. Some geologists think that the changing environmental conditions of the Cryogenian are what triggered the evolution of large and complex life.
There have been three major glaciations during the Phanerozoic (the past 540 Ma). These include the Andean/Saharan (recorded in rocks of South America and Africa), the Karoo (named for rocks in southern Africa), and the Cenozoic glaciations. The Karoo was the longest of the Phanerozoic glaciations, persisting for much of the time that the supercontinent Gondwana was situated over the South Pole (~360 to 260 Ma). Glaciers covered large parts of Africa, South America, Australia, and Antarctica. This widespread glaciation, across continents that are now far apart, was an important component of Alfred Wegener’s evidence for continental drift. Unlike the Cryogenian glaciations, the Andean/Saharan, Karoo, and Cenozoic glaciations only affected parts of Earth. During Karoo times, for example, what is now North America was near the equator and remained unglaciated.
Earth was warm and essentially unglaciated throughout the Mesozoic. Although there may have been some alpine glaciers at this time, there is no evidence for them preserved in the geologic record. The dinosaurs, which dominated terrestrial habitats during the Mesozoic, did not have to endure icy conditions.
17.4.1 Cenozoic Glaciations
A warm climate persisted into much of the Cenozoic; there is evidence that the Paleocene (from about 50 to 60 Ma) was the warmest part of the Phanerozoic since the Cambrian (Figure 17.35).
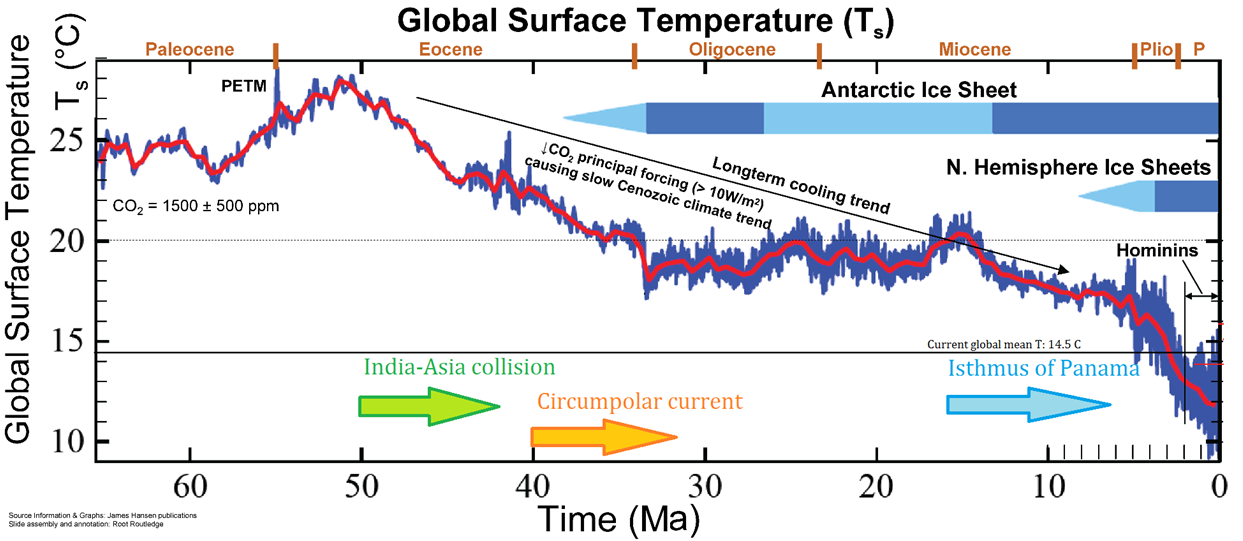) and Makiko Sato & James Hansen (_2012), _including data from Zachos et al (2008) _[_view original_](http://www.columbia.edu/~mhs119/Sensitivity+SL+CO2/)](figures/17-glaciation/figure-17-35.png)
Figure 17.35: Global temperature trends over the past 65 Ma (the Cenozoic). From the end of the Paleocene to the height of the Pleistocene glaciation, global average temperature dropped by about 14°C. _ Source: Joyce McBeth (2018) CC BY 4.0. Modified after Steven Earle (2015) CC BY 4.0 (view source) and Makiko Sato & James Hansen (_2012), including data from Zachos et al (2008) view original
A number of tectonic events during the Cenozoic have contributed to persistent and significant planetary cooling from 50 Ma to near the present. For example, the collision of the Indian plate with the Eurasian plate and the formation of the Himalayan range and the Tibetan Plateau. Mountain building events such as the formation of the Himalayas are followed by weathering and erosion of the uplifted rocks. Higher than normal global rates of silicate mineral weathering associated with mountain building, especially weathering of feldspar, leads to a decrease in carbon dioxide concentrations in the atmosphere. This contributes to global climate cooling.
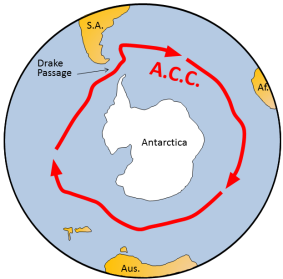](figures/17-glaciation/figure-17-36.png)
Figure 17.36: The Antarctic Circumpolar Current (red arrows) prevents warm water from the rest of Earth’s oceans from reaching Antarctica. Source: Steven Earle (2015) CC BY 4.0 view source
At 40 Ma, ongoing plate motion widened the narrow gap between South America and Antarctica, resulting in the opening of the Drake Passage. This allowed for unrestricted west-to-east flow of water around Antarctica: the Antarctic Circumpolar Current (Figure 17.36), which effectively isolated the Southern Ocean from the warmer waters of the Pacific, Atlantic, and Indian Oceans. The region cooled significantly, and by 35 Ma (Oligocene) glaciers had started to form on Antarctica.
Global temperatures remained relatively steady during the Oligocene and early Miocene, and the Antarctic glaciation waned during that time. At around 15 Ma, subduction-related volcanism between central and South America created the land connection between North and South America, preventing water from flowing between the Pacific and Atlantic Oceans. This further restricted ocean currents that transfer heat from the tropics to the poles, leading to cooling and advance of the Antarctic glaciation.
The expansion of the Antarctic ice sheet increased reflection of solar radiation at the Earth’s surface and promoted a positive feedback loop of further cooling. With more reflective glacial ice, there was more cooling, leading to accumulation of more ice, and so on. By the Pliocene (~5 Ma) ice sheets had started to grow in North America and northern Europe. The most intense part of the current glaciation — and the coldest climate conditions of the current glaciation — has been during the past million years (the last third of the Pleistocene).
The Pleistocene Epoch of the Cenezoic Era (2.58 Ma to 0.126 Ma), is also known as the Ice Age, Pleistocene Glaciation, or Quaternary Glaciation. The Pleistocene has been characterized by temperature fluctuations over a range of almost 10°C on time scales of 40,000 to 100,000 years. These temperature variations have corresponding with expansion and contraction of ice sheets. The temperature variations are attributed to subtle changes in Earth’s orbit, tilt, and wobble. These cyclical changes are called Milankovitch cycles. Over the past million years, the glaciation cycles have cycled over every 100,000 years, approximately (Figure 17.37).
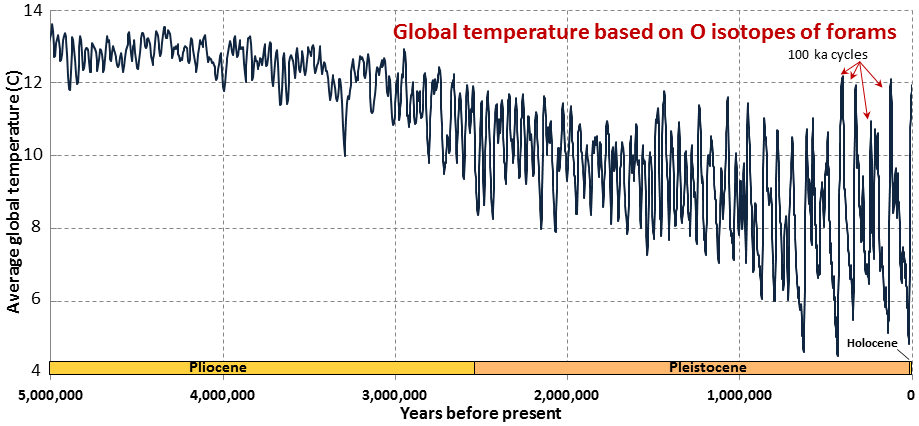. Data from Lisiecki and Raymo (2005). [Access the data](http://www.lorraine-lisiecki.com/stack.html)_](figures/17-glaciation/figure-17-37.png)
Figure 17.37: Foram oxygen isotope record for the past 5 million years based on O isotope data from sea-floor sediments Source: Steven Earle (2015) CC BY 4.0 view source. Data from Lisiecki and Raymo (2005). Access the data
17.4.2 The Wisconsinian Glaciation
The __Wisconsinan Glaciation __was the last major continental glaciation in _ North America _(from 150-50 ka). During the Wisconsinan, all of Canada and a small portion of the northern United States was covered with continental glaciers (Figure 17.38). The massive Laurentide Ice Sheet covered most of eastern Canada, as far west as the Rockies, and the smaller Cordilleran Ice Sheet covered most of the western region of present day BC and the Yukon Territory. At various other glacial peaks during the Pleistocene and Pliocene, the ice extent was similarly distributed over North America, and in some cases, was even more extensive. The combined Laurentide and Cordilleran Ice Sheets were comparable in volume to the current Antarctic Ice Sheet.
<br>_](figures/17-glaciation/figure-17-38.png)
Figure 17.38: Extent of northern hemisphere ice sheets near the peak of the Wisconsinan Glaciation (grey shading). Interglacial ice is shown in black. Source: Hannes Grobe (2008) CC BY 3.0 view source
Exercise: Pleistocene Glacial and Interglacial Periods
Figure 17.39 shows the past 500,000 years of the data set used in Figure 17.37. The last five glacial periods are marked with snowflakes. The most recent glaciation, which peaked at around 20 ka, is known as the Wisconsinan Glaciation. Describe the nature of temperature change that followed each of these glacial periods.
The current interglacial period (Holocene) is marked with an H. Point out the previous five interglacial periods.
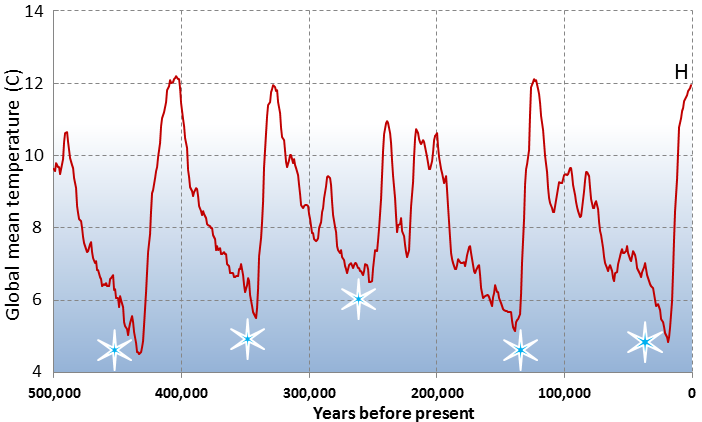), using data from Lisiecki and Raymo (2005) [Access the data](http://www.lorraine-lisiecki.com/stack.html)_](figures/17-glaciation/figure-17-39.png)
Figure 17.39: Global mean temperatures over the last 500,000 years. Source: Steven Earle (2015) CC BY 4.0 (view source), using data from Lisiecki and Raymo (2005) Access the data
17.5 Summary
The topics covered in this chapter can be summarized as follows:
17.5.1 Types of Glaciers
The two main types of glaciers are continental glaciers, which are very large and cover large parts of continents (e.g. the Antarctic Ice Sheet), and alpine glaciers, which occupy mountainous regions. Ice accumulates at higher elevations — above the equilibrium line — where the snow that falls in winter does not all melt in summer. In continental glaciers, ice flows outward from where it is thickest. In alpine glaciers, ice also flows from thicker to thinner regions in the glacier, obeying the law of gravity. At depth in glacier ice, flow occurs through internal deformation, but glaciers that have liquid water at their base can also flow by basal sliding. Crevasses form in the rigid surface ice in places where the lower plastic ice is changing flow rate or shape as it moves over the underlying topography.
17.5.2 Glacial Erosion
Glaciers are important agents of erosion. Continental glaciers tend to erode land surface into flat plains, while alpine glaciers create a wide variety of different erosional features. The key feature of alpine glacial erosion is the U-shaped valley. Arêtes are sharp ridges that form between two valleys, and horns form where a mountain is glacially eroded on at least three sides. Since tributary glaciers do not erode as deeply as main-valley glaciers, hanging valleys exist where the two meet. On a smaller scale, both types of glaciers form roche moutonnées, d glacial grooves, and striae.
17.5.3 Glacial Deposits
Glacial deposits form as materials are transported and deposited in a variety of different ways in a glacial environment. Sediments that are moved and deposited directly by ice are known as till. Till deposits left at the edges of the glacier as it recedes are known as moraines. Till can also form features such as drumlins (oval-shaped elongated hills) and kettle lakes. Glaciofluvial sediments are deposited by glacial streams, either forming eskers or large proglacial plains known as sandurs. Glaciolacustrine and glaciomarine sediments originate within glaciers and are deposited in lakes and oceans, respectively.
17.5.4 Glaciations over Earth’s History
There have been many glaciations in Earth’s past, the oldest known starting about 2.4 Ga. The late Proterozoic “Snowball Earth” glaciations were thought to be sufficiently intense to affect the entire planet. The Pleistocene Glaciation was a series of glacial events over the past 2.85 Ma. The periodicity of glaciations in the Pleistocene is related to subtle changes in Earth’s orbital characteristics (Milankovitch cycles), which are exaggerated by positive climate feedback processes. North America was most recently glaciated during the Wisconsinan Glaciation, from 150-50 ka.
17.6 Chapter Review Questions
What feature of a continental glacier causes it to flow?
Explain how alpine glacier ice flows.
What does the equilibrium line represent in a glacier?
Which of the following is more important to the growth of a glacier: very cold winters or relatively cool summers? Why?
Describe the relative rates of ice flow, and why the rate distributions are the way they are, within the following parts of a glacier: (a) the bottom versus the top and (b) the edges versus the middle.
Figure 17.40 shows thrust faults in the leading edge of the Athabasca glacier in Alberta. Find the thrust faults in the glacier. Note: they are harder to distinguish in this image than in Figure 17.12 because they don’t have sediments along the faults.
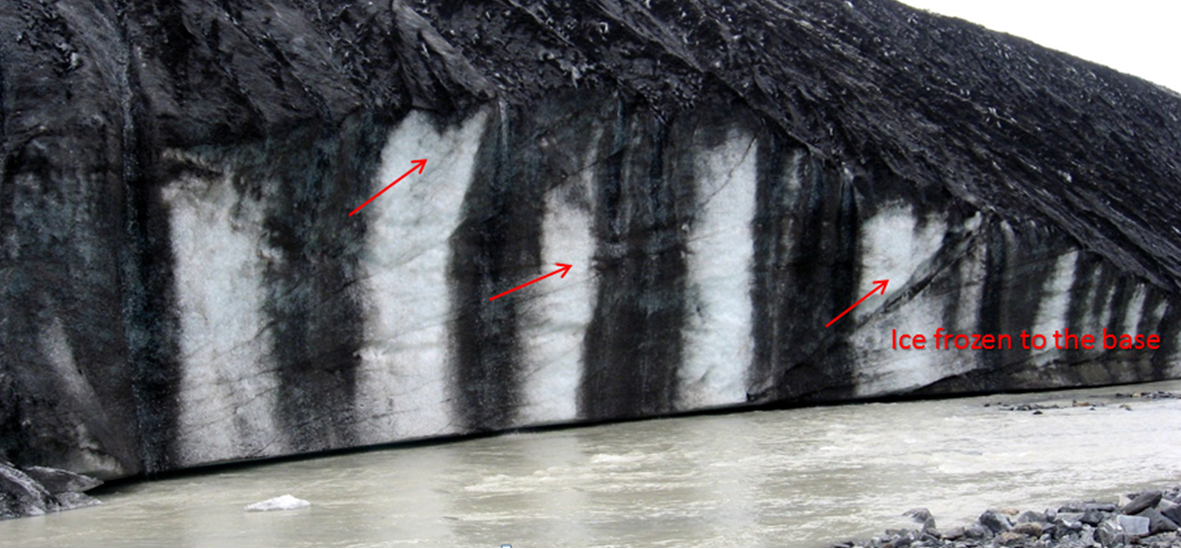_](figures/17-glaciation/figure-17-40.jpg)
Figure 17.40: Edge of the Athabasca glacier in Alberta. Source: Steven Earle (2015) CC BY 4.0 view source
What ice conditions are necessary for basal sliding to take place?
What sources of heat can lead to melting and/or water accumulation at the base of a glacier?
Why do glaciers carve U-shaped valleys, and how does a hanging valley form? How is a river valley different than a glacial valley?
A horn is typically surrounded by cirques. What is the minimum number of cirques you would expect to find around a horn?
A drumlin and a roche moutonnée are both streamlined glacial erosion features. How do they differ in shape? How can you determine the direction of glacial flow from their shapes?
What are drop stones, and under what circumstances are they likely to form?
What types of glacial sediments are likely to be sufficiently permeable to make good aquifers?
Why are the Cryogenian glaciations called Snowball Earth?
Earth cooled dramatically from the end of the Paleocene until the Holocene. Describe some of the geological events that contributed to this cooling.
When and where was the first glaciation of the Cenozoic?
Describe the extent of the Laurentide Ice Sheet during the height of the last Pleistocene glacial period.
Four examples of glacial sediments are shown in Figure 17.41 below. Describe the important characteristics (e.g., sorting, layering, grain-size range, grain shape, sedimentary structures) of each, and give each a name (choose from glaciofluvial, glaciolacustrine, lodgement till, ablation till, and glaciomarine).
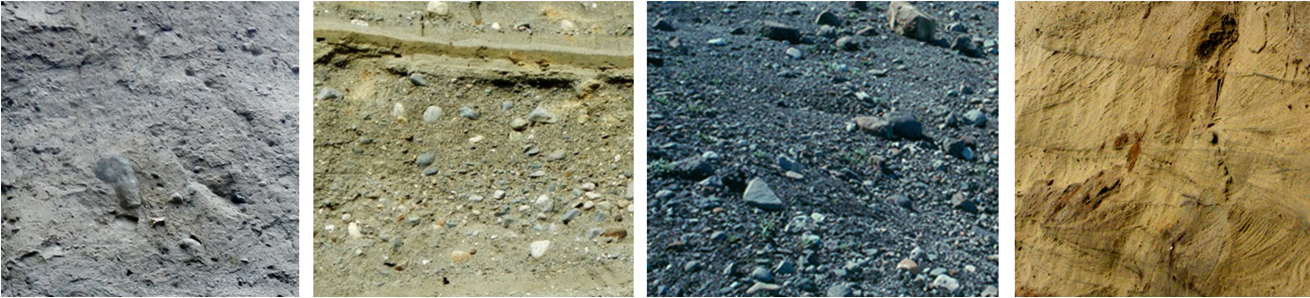_](figures/17-glaciation/figure-17-41.png)
Figure 17.41: Examples of glacial sediments. Source: Steven Earle (2015) CC BY 4.0 view source
17.7 Answers to Chapter Review Questions
Continental glaciers flow from the areas where the ice is thickest (and therefore at the highest elevation) toward areas (at the margins) where the ice is thinnest. Ice thickness tends to be related to the rate of ice accumulation.
Alpine glaciers flow by gravity.
The equilibrium line represents the boundary between the area where ice is accumulating (typically at high elevations), and where it is being depleted (mostly by melting). Above the equilibrium line more snow accumulates in winter than can melt in summer so the glacier is always covered in snow. Below the equilibrium line the snow cover is lost by the end of summer.
Relatively cool summers are more important because that controls how much snow will melt in the summer. In many situations very cold winters are associated with less snow accumulation than just cold winters.
- The ice at the bottom of a glacier flows more slowly than that at the top. In fact if the glacier is frozen to its base the lowermost ice might not be moving at all. (b) The edges also flow more slowly than the middle because there is more friction there between the ice and the valley walls.
Basal sliding will take place when the bed of the glacier is warm enough for water to be liquid. The water will act as a lubricant to allow the ice to flow.
Glaciers carve U-shaped valleys because they are relatively wide (compared with rivers) and most of the erosion takes place at the base rather than the sides. A hanging valley forms where a tributary glacier joins a larger glacier and where the larger glacier has eroded a deeper valley.
There must be at least three cirques to form a horn. In most cases there wouldn’t be room for more than four.
A drumlin is relatively steep at the up-ice end and streamlined at the down-ice end. A roche moutonée is streamlined at the up-ice end and jagged at the down-ice end where plucking has taken place.
Drop stones are large clasts that are present with lacustrine or marine glacial sediments. They form when coarse material drops from melting icebergs.
Glaciofluvial sediments (sand or sand and gravel) are likely to be sufficiently permeable to make good aquifers.
The Cryogenian glaciations are called Snowball Earth because it is thought that freezing conditions affected the entire planet and that the oceans were frozen over, even at the equator.
The cooling from the end of the Paleocene until the Holocene was related to the formation of mountains including the Himalayas, the Rockies, and the Andes; the opening of the Drake Passage; the development of Antarctic Circumpolar Current; and the closing of the Isthmus of Panama.
The first glaciation of the Cenozoic took place in Antarctica during the Oligocene (around 30 Ma).
At the height of the last glaciation, the Laurentide Ice Sheet covered almost all of Canada and extended south into the United States as far as Wisconsin.
From left to right, the sediments are:
- Lodgement till: Poorly sorted, clay to boulders, no layering, large clasts not well rounded, no structures.
- Glaciofluvial sand and gravel: Bedded, some fine beds of sand, and some much coarser beds. Clasts are relatively well rounded.
- Ablation till: Poorly sorted, pebbles to boulders, no layering, large angular clasts, no apparent structures.
- Glaciofluvial sand: Sand- and silt-sized, well-sorted, bedded and cross-bedded.
17.8 References
Hansen, J. E., and Sato, M. (2012). Climate Sensitivity Estimated From Earth’s Climate History. Read the paper
Lisiecki, L. E., and M. E. Raymo (2005). A Pliocene-Pleistocene stack of 57 globally distributed benthic d18O records. Paleoceanography, 20, PA1003. doi:10.1029/2004PA001071. View PDF
Zachos, J. C., Dickens, G. R., and Zeebe, R. E. (2008). An early Cenozoic perspective on greenhouse warming and carbon-cycle dynamics. Nature 541, 279-283. doi:10.1038/nature06588